22 Chapter 22: Passive and Active Transport
Lisa Limeri
Learning Objectives
By the end of this section, students will be able to:
- Explain how the size, polarity, and charge of a molecule affects its ability to cross a phospholipid membrane.
- Compare the processes of diffusion, osmosis, and facilitated diffusion, and provide biological examples that illustrate each process.
- Define passive, active, and secondary active transport and explain the role of channels, carriers, pumps, and co-transporters in transport.
Introduction
The plasma membrane, also called the cell membrane, has many functions. The most basic function is to define the cell’s borders and keep the cell functional. The plasma membrane is selectively permeable. This means that the membrane allows some materials to freely enter or leave the cell, while other materials cannot move freely, but require a specialized structure, and occasionally, even energy investment for crossing.
Cell Membrane Components and Structure
A cell’s plasma membrane defines the cell, outlines its borders, and determines the nature of its interaction with its environment. Cells exclude some substances, take in others, and excrete still others, all in controlled quantities. The plasma membrane must be very flexible to allow certain cells, such as red and white blood cells, to change shape as they pass through narrow capillaries. These are the more obvious plasma membrane functions. In addition, the plasma membrane’s surface carries markers that allow cells to recognize one another, which is vital for tissue and organ formation during early development, and which later plays a role in the immune response’s “self ” versus “non-self” distinction.
The fluid mosaic model (Fig 22.1) describes the plasma membrane structure as a mosaic of components—including phospholipids, cholesterol, proteins, and carbohydrates—that gives the membrane a fluid character. Plasma membranes range from 5 to 10 nm in thickness. For comparison, human red blood cells, visible via light microscopy, are approximately 8 μm wide, or approximately 1,000 times wider than a plasma membrane. The membrane does look a bit like a sandwich (Figure 22.1).
A plasma membrane’s principal components are lipids (phospholipids and cholesterol), proteins, and carbohydrates attached to some of the lipids and proteins. A phospholipid is a molecule consisting of glycerol, two fatty acids, and a phosphate-linked head group. Cholesterol, another lipid comprised of four fused carbon rings, is situated alongside the phospholipids in the membrane’s core. Carbohydrates are present only on the plasma membrane’s exterior surface and are attached to proteins, forming glycoproteins, or attached to lipids, forming glycolipids. The protein, lipid, and carbohydrate proportions in the plasma membrane vary with cell type, but for a typical human cell, protein accounts for about 50% of the composition by mass, lipids (of all types) account for about 40%, and carbohydrates comprise the remaining 10%. However, protein and lipid concentration varies with different cell membranes.
Phospholipids
The membrane’s main fabric comprises amphiphilic phospholipid molecules. The hydrophilic or “water-loving” areas of these molecules (which look like a collection of balls in an artist’s rendition of the model) (Figure 22.2) are in contact with the aqueous fluid both inside and outside the cell. Hydrophobic, or “water-fearing” molecules, tend to be non-polar. They generally do not interact with polar molecules. When placed in water, hydrophobic molecules tend to form a ball or cluster due to the hydrophobic effect. The phospholipids’ hydrophilic regions form hydrogen bonds with water and other polar molecules on both the cell’s exterior and interior. Thus, the membrane surfaces that face the cell’s interior and exterior are hydrophilic. In contrast, the cell membrane’s interior is hydrophobic and will not interact with water. Therefore, phospholipids form an excellent two-layer cell membrane that separates fluid within the cell from the fluid outside the cell.
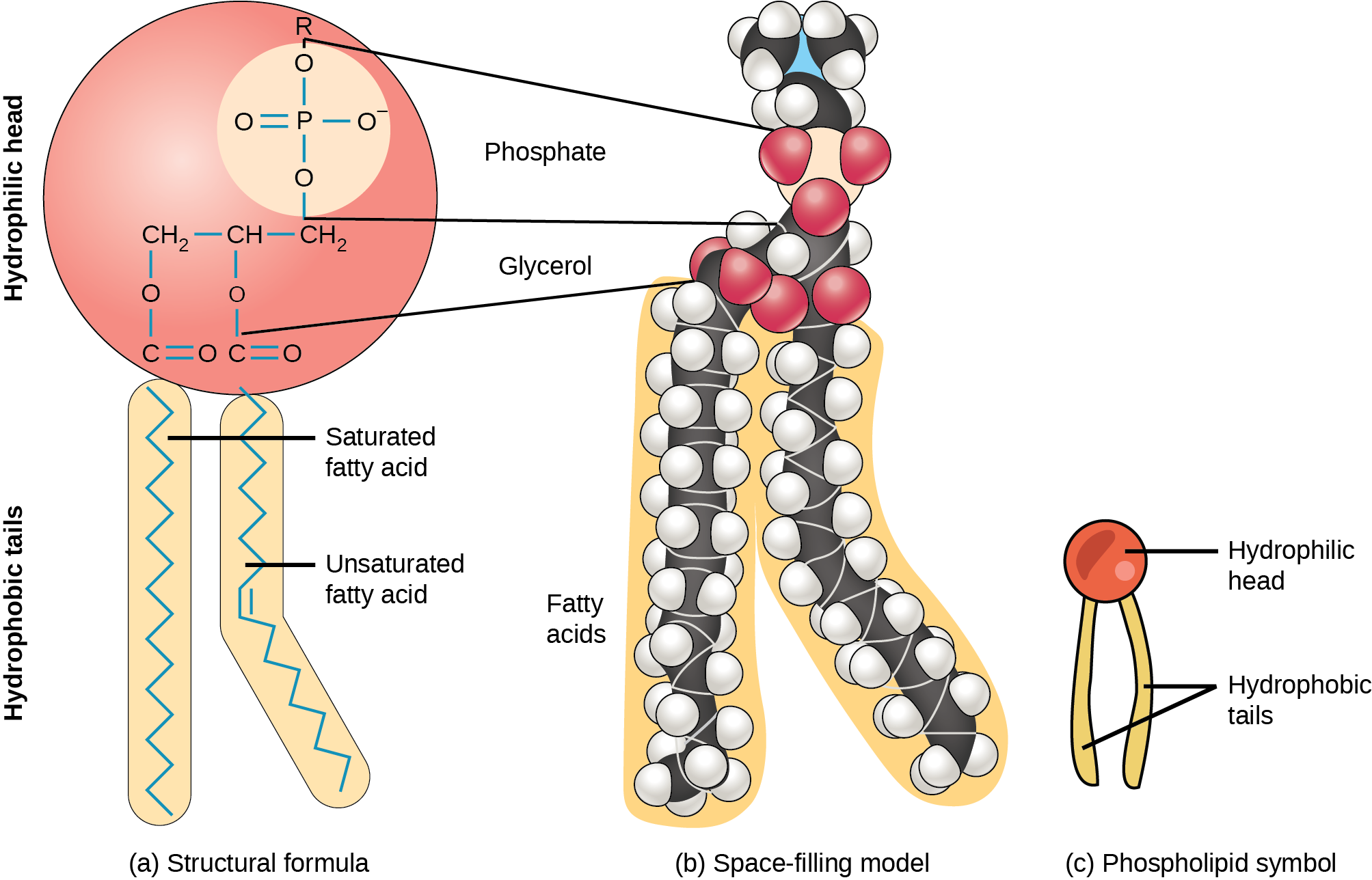
This characteristic is vital to the plasma membrane’s structure because, in water, phospholipids arrange themselves with their hydrophobic tails facing each other and their hydrophilic heads facing out. In this way, they form a lipid bilayer—a double layered phospholipid barrier that separates the water and other materials on one side from the water and other materials on the other side.
Proteins
Proteins comprise the plasma membranes’ second major component. Integral proteins, as their name suggests, integrate completely into the membrane structure, and their hydrophobic membrane-spanning regions interact with the phospholipid bilayer’s hydrophobic region (Figure 22.3). Single-pass integral membrane proteins usually have a hydrophobic transmembrane segment. Some span only part of the membrane—associating with a single layer—while others stretch from one side to the other, and are exposed on either side. Up to 12 single protein segments comprise some complex proteins, which are extensively folded and embedded in the membrane (Figure 22.3). This protein type has a hydrophilic region or regions, and one or several mildly hydrophobic regions. This arrangement of protein regions orients the protein alongside the phospholipids, with the protein’s hydrophobic region adjacent to the phospholipids’ tails and the protein’s hydrophilic region or regions protruding from the membrane and in contact with the cytosol or extracellular fluid.
Peripheral proteins are on the membranes’ exterior and interior surfaces, attached either to integral proteins or to phospholipids (Figure 22.3). Peripheral proteins, along with integral proteins, may serve as enzymes, as structural attachments for the cytoskeleton’s fibers, or as part of the cell’s recognition sites. Scientists sometimes refer to these as “cell-specific” proteins. The body recognizes its own proteins and attacks foreign proteins associated with invasive pathogens.
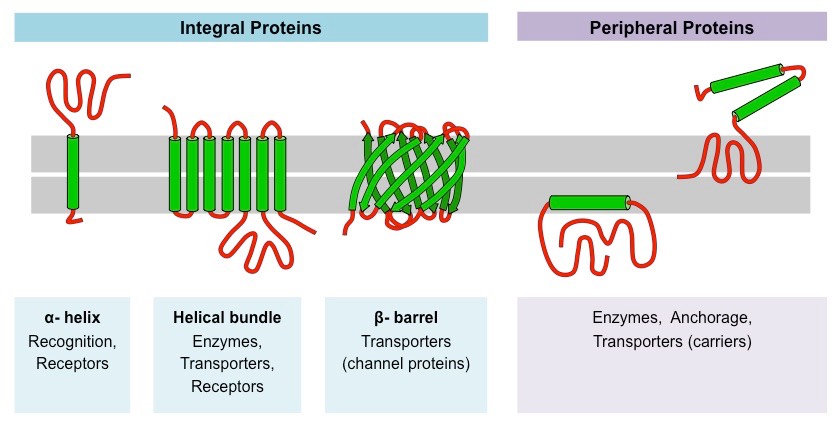
Carbohydrates
Carbohydrates are the third major plasma membrane component. They are always on the cells’ exterior surface and are bound either to proteins (forming glycoproteins) or to lipids (forming glycolipids) (Figure 22.1). Along with peripheral proteins, carbohydrates form specialized sites on the cell surface that allow cells to recognize each other. These sites have unique patterns that allow for cell recognition, much the way that the facial features unique to each person allow individuals to recognize them. This recognition function is very important to cells, as it allows the immune system to differentiate between body cells (“self”) and foreign cells or tissues (“non-self”). Similar glycoprotein and glycolipid types are on the surfaces of viruses and may change frequently, preventing immune cells from recognizing and attacking them.
Passive Transport
The most direct forms of membrane transport are passive. In passive transport, substances move from an area of higher concentration to an area of lower concentration. Passive transport is a naturally occurring phenomenon and does not require the cell to exert any of its energy to accomplish the movement. A physical space in which there is a single substance concentration range has a concentration gradient. Molecules will tend to move from areas of higher concentration to lower concentration, often called “down” the concentration gradient. This does not require the input of energy, and is called passive transport.
Diffusion
Diffusion is one type of passive transport. Diffusion occurs when molecules moves from a high concentration to a low concentration area until the concentration is equal across a space. You are familiar with diffusion of substances through the air. For example, think about someone opening a bottle of ammonia in a room filled with people. The ammonia gas is at its highest concentration in the bottle. Its lowest concentration is at the room’s edges. The ammonia vapor will diffuse, or spread away, from the bottle, and gradually, increasingly more people will smell the ammonia as it spreads. Diffusion costs no energy. On the contrary, concentration gradients are a form of potential energy, which dissipates as the gradient is eliminated.
Plasma membranes must allow certain substances to enter and leave a cell, and prevent some harmful materials from entering and some essential materials from leaving. In other words, plasma membranes are selectively permeable—they allow some substances to pass through, but not others. If they were to lose this selectivity, the cell would no longer be able to sustain itself, and it would be destroyed. Recall that plasma membranes are amphiphilic: they have hydrophilic and hydrophobic regions. This characteristic helps move some materials through the membrane and hinders the movement of others. Nonpolar (a.k.a. lipid-soluble) molecules with a low molecular weight (i.e., are small) can easily slip through the membrane’s hydrophobic lipid core. Substances such as the fat-soluble vitamins A, D, E, and K readily pass through the plasma membranes in the digestive tract and other tissues. Fat-soluble drugs and hormones also gain easy entry into cells and readily transport themselves into the body’s tissues and organs. Oxygen and carbon dioxide molecules have no charge and pass through membranes by simple diffusion. In summary, molecules that are small and lipid-soluble (hydrophobic) can pass directly through the membrane down their concentration gradient without any facilitation by proteins or input of energy. This is described as simple diffusion.
Reading Question #1
Which of the following molecules can directly diffuse through a plasma membrane?
A. A large, polar molecule.
B. A small, non-polar molecule.
C. A large, charged molecule.
D. A small, charged molecule.
Facilitated Transport
Polar and charged substances present problems for the membrane. While some polar molecules connect easily with the cell’s outside, they cannot readily pass through the plasma membrane’s lipid core. Additionally, while small ions could easily slip through the spaces in the membrane’s mosaic, their charge prevents them from doing so. Ions such as sodium, potassium, calcium, and chloride must have special means of penetrating plasma membranes. Molecules that cannot pass directly through the membrane require the help of transmembrane proteins to transport themselves across plasma membranes.
In facilitated transport, materials passively transport across the plasma membrane with the help of membrane proteins. A concentration gradient exists that would allow these materials to pass into the cell without expending cellular energy. However, these materials are polar or charged molecules that the cell membrane’s hydrophobic parts repel, or too large to fit through the membrane without aid. Facilitated transport proteins shield these materials from the membrane’s repulsive force, or create a large enough passageway, allowing them to diffuse into the cell.
The integral proteins involved in facilitated transport are transport proteins, that come in two types: channels or carriers. Both types of transport proteins are transmembrane proteins and are specific to transporting a particular molecule. Channel proteins have hydrophilic domains exposed to the intracellular and extracellular fluids. In addition, they have a hydrophilic channel through their core that provides a hydrophilic opening through the hydrophobic lipid bilayer interior (Figure 22.4). Passage through the channel allows polar or charged compounds to avoid the plasma membrane’s nonpolar central layer that would otherwise slow or prevent their entry into the cell. Aquaporins are channel proteins that allow water to pass through the membrane at a very high rate. Channel proteins are either open at all times or they are “gated,” which controls the channel’s opening. When a particular ion attaches to the channel protein it may control the opening, or other mechanisms or substances may be involved. In some tissues, sodium and chloride ions pass freely through open channels; whereas, in other tissues a gate must open to allow passage. An example of this occurs in the kidney, where there are both channel forms in different parts of the renal tubules.
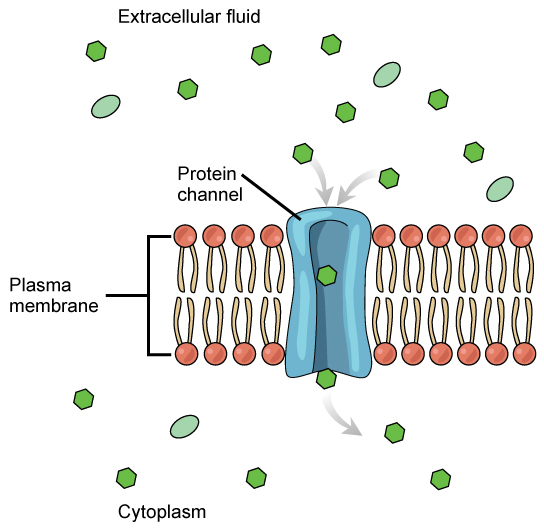
Carrier proteins facilitate transport of molecules across a membrane by binding to a molecule, which triggers a change of its own shape and results in moving the bound molecule from one side of the membrane to the other (Figure 22.5). The key difference between a channel and carrier protein is that a channel protein’s shape is static whereas a carrier protein changes shape as it facilitates transport. Each carrier protein is specific to one substance, and there are a finite number of these proteins in any membrane. This can cause problems in transporting enough material for the cell to function properly. When all of the proteins are bound to their ligands, they are saturated and the rate of transport is at its maximum. Increasing the concentration gradient at this point will not result in an increased transport rate. An example of this process occurs in the kidney. In one part, the kidney filters glucose, water, salts, ions, and amino acids that the body requires. This filtrate, which includes glucose, then reabsorbs in another part of the kidney. Because there are only a finite number of carrier proteins for glucose, if more glucose is present than the proteins can handle, the excess is not transported and the body excretes this through urine. This is why a common symptom of diabetes is urine that smells sweet – because there is glucose in it.
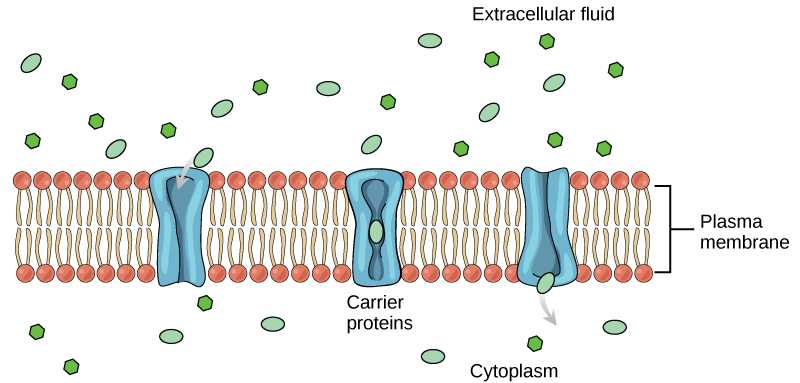
Reading Question #2
What is the primary distinction between simple diffusion and facilitated diffusion?
A. Facilitated diffusion transports more molecules simultaneously than simple diffusion.
B. Facilitated diffusion transports more complex molecules than simple diffusion.
C. Facilitated diffusion involves transmembrane proteins whereas simple diffusion does not.
D. Facilitated diffusion requires energy whereas simple diffusion does not.
Active Transport
Some cells require larger amounts of specific substances. They must have a way of obtaining these materials from extracellular fluids. This may happen passively, as certain materials move back and forth, or the cell may have special mechanisms that facilitate transport. Some materials are so important to a cell that it spends some of its energy, hydrolyzing adenosine triphosphate (ATP), to obtain these materials. Red blood cells use some of their energy doing just that. Most cells spend the majority of their energy to maintain an imbalance of sodium and potassium ions between the cell’s interior and exterior, as well as on protein synthesis.
Active transport mechanisms require the cell’s energy, usually in the form of adenosine triphosphate (ATP). If a substance must move into the cell against its concentration gradient—that is, if the substance’s concentration inside the cell is greater than its concentration in the extracellular fluid (and vice versa)—the cell must use energy to move the substance. Some active transport mechanisms move small-molecular weight materials, such as ions, through the membrane. Other mechanisms transport much larger molecules.
Electrochemical Gradients
We have discussed simple concentration gradients—a substance’s differential concentrations across a space or a membrane—but in living systems, gradients are more complex. Because ions move into and out of cells and because cells contain proteins that do not move across the membrane and are mostly negatively charged, there is also an electrical gradient, a difference of charge, across the plasma membrane. The interior of living cells is electrically negative with respect to the extracellular fluid in which they exist, and at the same time, cells have higher concentrations of potassium (K+) and lower concentrations of sodium (Na+) than the extracellular fluid. Thus in a living cell, the concentration gradient of Na+ tends to drive it into the cell, and its electrical gradient (a positive ion) also drives it inward to the negatively charged interior. However, the situation is more complex for other elements such as potassium. The electrical gradient of K+, a positive ion, also drives it into the cell, but the concentration gradient of K+ drives K+ out of the cell (Figure 22.6). We call the combined concentration gradient and electrical charge that affects an ion its electrochemical gradient.
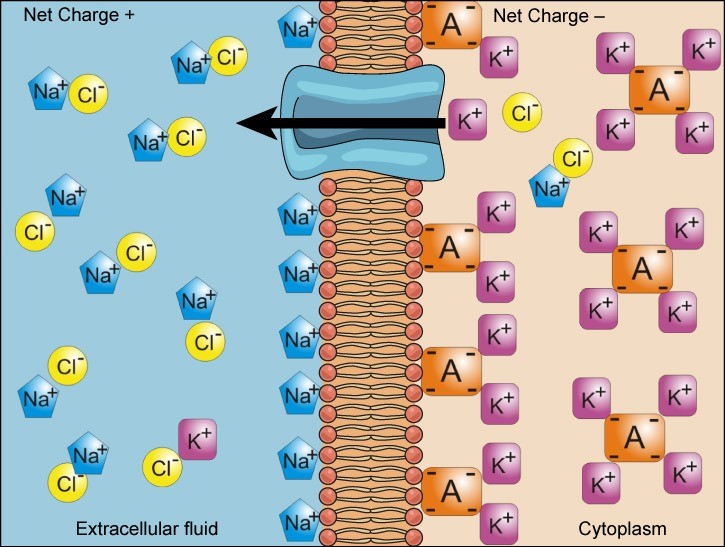
Reading Question #3
What is the primary distinction between facilitated diffusion and active transport?
A. Active transports moves multiple molecules simultaneously whereas facilitated diffusion only moves one molecule.
B. Active transport transports more complex molecules than facilitated diffusion.
C. Facilitated diffusion involves transmembrane proteins whereas active transport does not.
D. Active transport requires energy whereas facilitated diffusion does not.
Moving Against a Gradient Requires Specialized Proteins
To move substances against a concentration or electrochemical gradient, the cell must use energy. This energy comes from ATP generated through the cell’s metabolism (we will learn how ATP is created in Unit 5!). Active transport proteins, called pumps, move ions or molecules against their electrochemical gradients. Active transport is required to maintain concentrations of ions and other substances that living cells require to be different from the external environment. A cell may spend much of its metabolic energy supply maintaining these processes. For example, a red blood cell uses most of its metabolic energy to maintain the imbalance between exterior and interior sodium and potassium levels that the cell requires.
There are three types of proteins that transporting materials across the cell membrane that cannot diffuse across it, collectively called transporters (Figure 22.7). A uniporter carries one specific ion or molecule. A symporter carries two different ions or molecules, both in the same direction. An antiporter carries two different ions or molecules, but in different directions. When these transporters are moving material against the electrochemical gradient, they require energy and are carrying out active transport. When they are moving material with the electrochemical gradient, they do not require energy and are carrying out facilitated diffusion.
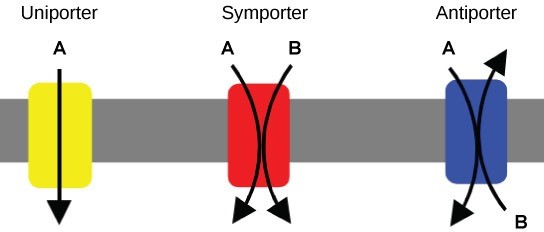
Two mechanisms exist for transporting small-molecular weight material and small molecules. Primary active transport moves ions across a membrane and creates a difference in charge across that membrane, which is directly dependent on ATP. Secondary active transport does not directly require ATP: instead, it is the movement of material due to the electrochemical gradient established by primary active transport.
Primary Active Transport
One of the most important pumps in animals cells is an antiporter that caries out active transport: the sodium potassium pump (Na+-K+ ATPase), which pumps sodium ions out of the cell and and potassium ions into the cell (Figure 22.8).

The sodium-potassium pump maintains the electrochemical gradient (and the correct concentrations of Na+ and K+) in living cells. The sodium-potassium pump moves K+ into the cell while moving Na+ out at the same time, at a ratio of three Na+ for every two K+ ions moved in. As a result of this pump, there are more sodium ions outside the cell than inside and more potassium ions inside than out. For every three sodium ions that move out, two potassium ions move in. This results in the interior being slightly more negative relative to the exterior. This difference in charge is important in creating the conditions necessary for many cellular functions, including secondary active transport (more on that next).
Reading Question #4
What is the main purpose of the sodium-potassium pump (Na+-K+ ATPase) in animal cells?
A. Maintain electrochemical gradients of sodium and potassium.
B. Remove excess potassium from inside cells.
C. Create a surplus of sodium inside cells.
D. Equalize the concentration of sodium and potassium inside and outside cells.
Secondary Active Transport (Co-transport)
The activity of the sodium-potassium pump creates conditions that allows secondary active transport to occur. As sodium ion concentrations build outside of the plasma membrane because of the primary active transport process, this creates an electrochemical gradient.
If a channel or transport protein exists to facilitate passage of sodium ions, they will move into the cell. This movement releases energy that is stored in the electrochemical gradient across the membrane. This energy can be harnessed to simultaneously move another molecule against its electrochemical gradient. (Figure 22.9). Many amino acids, as well as glucose, enter a cell this way. This secondary process also stores high energy hydrogen ions in the mitochondria of plant and animal cells in order to produce ATP. The potential energy that accumulates in the stored hydrogen ions translates into kinetic energy as the ions surge through the channel protein ATP synthase, and that energy then converts ADP into ATP.
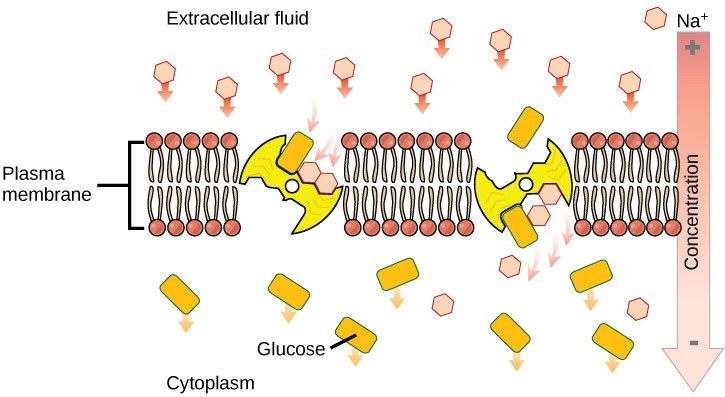
Reading Question #5
What is the main distinction between primary and secondary active transport?
A. Secondary active transport moves more complex molecules than primary active transport.
B. Secondary active transport moves more molecules simultaneously than primary active transport.
C. Primary active transport requires energy whereas primary active transport does not.
D. Primary active transport uses ATP as the direct source of energy whereas secondary active transport uses an electrochemical gradient as the direct source of energy.
Acknowledgements and references
Text and figures adapted from the following sources:
Clark, M.A., Douglas, M., and Choi, J. (2018, March 28). Biology 2e. OpenStax. Retrieved from https://openstax.org/books/biology-2e/pages/1-introduction
École Polytechnique Fédérale de Lausanne. (2019, May 23). Producing electricity at estuaries using light and osmosis. ScienceDaily. Retrieved from www.sciencedaily.com/releases/2019/05/190523111359.htm
Boundless, LibreTexts. 5.7: Passive Transport – Facilitated Transport, shared under a CC BY-SA 4.0 license. https://bio.libretexts.org/Bookshelves/Introductory_and_General_Biology/Book%3A_General_Biology_%28Boundless%29/05%3A_Structure_and_Function_of_Plasma_Membranes/5.07%3A_Passive_Transport_-__Facilitated_Transport