7 Chapter 7: Meiosis and Sexual Reproduction: Meiosis I
Lisa Limeri; Joshua Reid; and Shifath Bin Syed
Learning Objectives
By the end of this section, you will be able to do the following:
- Explain why the segregation of homologous chromosomes in meiosis I leads to a reduction in ploidy.
- Explain how meiosis I can lead to genetic diversity in gametes.
- Distinguish between sister chromatids and homologous chromosomes.
Introduction
The ability to reproduce is a fundamental characteristic of all organisms: Hippopotamuses give birth to hippopotamus calves; Joshua trees produce seeds from which Joshua tree seedlings emerge; and adult flamingos lay eggs that hatch into flamingo chicks (Fig 7.1).
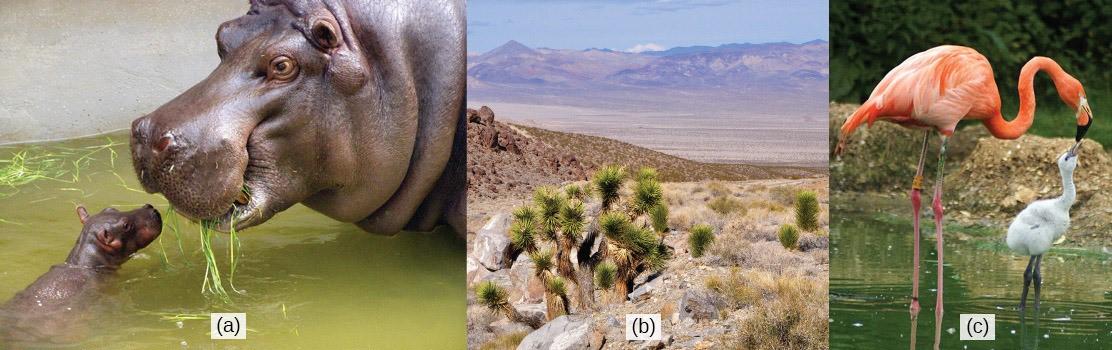
Although many unicellular organisms and a few multicellular organisms can produce genetically identical clones of themselves through asexual reproduction, many single-celled organisms and most multicellular organisms reproduce through sexual reproduction. In sexual reproduction, two parents each produce gametes, and the fusion of two gametes forms a zygote, which develops into a genetically unique organism. Gametes are produced by a type of cell division called meiosis. Sexual reproduction has been evolutionarily successful because it introduces variation into offspring. Most eukaryotic organisms, both multicellular and unicellular, can or must employ some form of meiosis and fertilization to reproduce.
Sexual Reproduction
Sexual reproduction was likely an early evolutionary innovation after the appearance of eukaryotic cells. It appears to have been very successful because most eukaryotes can reproduce sexually, and, in many animals, it is the only mode of reproduction. And yet, scientists also recognize some real disadvantages to sexual reproduction. On the surface, creating offspring that are genetic clones of the parent appears to be a better system. If the parent organism successfully occupies a habitat, offspring with the same traits should be similarly successful. There is also the obvious benefit of an organism that can produce offspring whenever circumstances are favorable by asexual budding, fragmentation, or by producing eggs asexually. These methods of reproduction do not require another organism of the opposite sex. Indeed, some organisms that lead a solitary lifestyle have retained the ability to reproduce asexually. In addition, in asexual populations, every individual is capable of reproduction. In sexual populations, the males are not producing the offspring themselves, so hypothetically, an asexual population could grow twice as fast.
However, multicellular organisms that exclusively depend on asexual reproduction are exceedingly rare. Why are meiosis and sexual reproductive strategies so common? These are important questions in biology. There are several possible explanations, one of which is that the variation that sexual reproduction creates among offspring is very important to the survival and reproduction of the population. Thus, on average, a sexually reproducing population will leave more descendants than an otherwise similar asexually reproducing population. The only source of variation in asexual organisms is mutation. Mutations during germline cell line formation are also the ultimate source of variation in sexually reproducing organisms. However, in contrast to mutations during asexual reproduction, mutations during sexual reproduction can be continually reshuffled from one generation to the next when different parents combine their unique genomes, and the genes are mixed into different combinations.
Reading Question #1
Why is sexual reproduction more common in multicellular organisms compared to asexual reproduction?
A. Sexual reproduction allows for the production of genetic clones.
B. Asexual reproduction requires two parents of the opposite sex.
C. Sexual reproduction results in increased genetic variation.
D. Asexual reproduction is faster than sexual reproduction.
The Red Queen Hypothesis
Genetic variation is the outcome of sexual reproduction, but why are endless variations necessary, even under seemingly stable environmental conditions? Enter the Red Queen hypothesis, first proposed by Leigh Van Valen in 1973 (Van Valen, 1973). The concept was named in reference to the Red Queen’s race in Lewis Carroll’s book, Through the Looking-Glass.
All species coevolve (evolve together) with other organisms. For example, predators evolve with their prey, and parasites evolve with their hosts. Each tiny advantage gained by favorable variation gives a species a reproductive edge over close competitors, predators, parasites, or even prey. However, the survival of any given genotype or phenotype in a population depends on the reproductive fitness of other genotypes or phenotypes within a given species. The only method that will allow a coevolving species to maintain its share of the resources is to continually improve its fitness (the capacity of the members to produce more reproductively viable offspring relative to others within a species). As one species gains an advantage, this increases the selection of the other species; they must also develop an advantage, or they will be outcompeted. No single species progresses too far ahead because genetic variation among the progeny of sexual reproduction provides all species with a rapid improvement mechanism. Species that cannot keep up become extinct. The Red Queen’s catchphrase was, “It takes all the running you can do to stay in the same place.” This is an apt description of coevolution between competing species.
Reading Question #2
What is the main advantage of sexual reproduction in coevolving species, according to the Red Queen hypothesis?
A. Genetic variation among offspring
B. Production of genetic clones
C. Faster reproduction compared to asexual reproduction
D. Avoiding competition with other species
Research Connection: Coevolution of Bats and Pepper Plants
The dispersal syndrome hypothesis posits that plants may select fruit traits (ex., size, color, scent) to match the sensory abilities (ex., vision, olfaction) of animals they want to attract (ex., effective seed dispersers). A recent study led by Dr. Sharlene Santana and Dr. Zofia Kaliszewska was one of the first published tests of bat-fruit coevolution. Their publication was based on data collected at La Selva Biological Station in Costa Rica. More than 50 Piper plants and three species of Carollia bats are located in this region. Carollia bats are critical dispersers of Piper plant seeds (University of Washington, 2021).
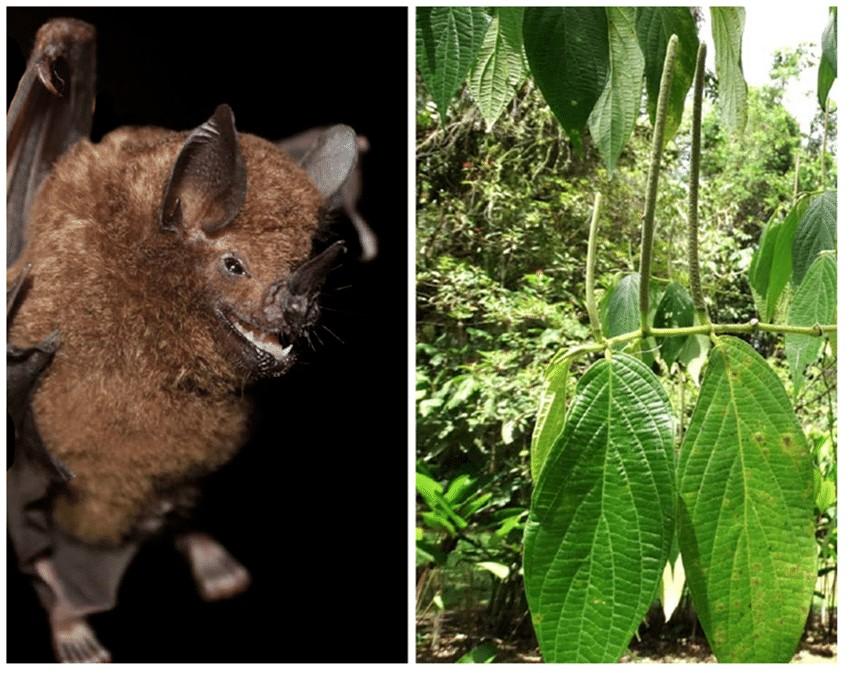
The research team searched for and collected ripe Piper fruits, fecal samples from Carollia bats, and recordings (video and audio) of Carollia bats’ echolocation and feeding behaviors. The research team then quantified the volatile organic compounds (VOCs) that make up the fragrance of each Piper fruit species. The research team also conducted behavioral experiments where unripe Piper fruit was spiked with common VOCs found in Piper plants (University of Washington, 2021).
After analyzing the Carollia bat data, the researchers found that all three species of Carollia consumed a lot of a select few Piper species and little of the others. Behavioral experiments provided clues as to why this occurred – bats preferred fruit samples spiked with 2-heptanol, a VOC found in the most highly consumed Piper species. Because bats fly at night, they rely on olfaction rather than sight to find ripe fruit. The research team’s findings suggest that Carollia bats use specific VOCs in the fruit’s fragrance to select ripe fruits from the specific Piper species that comprise most of their diet (University of Washington, 2021).
Meiosis
Each eukaryote species has a characteristic number of chromosomes in the nuclei of its cells. Human body (somatic) cells have 46 chromosomes, while human gametes (sperm or eggs) have 23 chromosomes each. A typical body cell contains two matched or homologous sets of chromosomes (one set from each biological parent)—a configuration known as diploidy. (Note: The letter n represents a single set of chromosomes; therefore, a diploid organism is designated 2n.) Human cells that contain one set of chromosomes are called gametes, or sex cells; these are eggs and sperm and are designated 1n, or haploid. Ploidy refers to the number of copies of each type of chromosome in a cell. Types of chromosomes are distinguished by the genes they code for. Copies of the same type of chromosome are homologous chromosomes. Some standard ploidy numbers are haploid (1n), diploid (2n), triploid (3n), and tetraploid (4n).
Upon fertilization, each gamete contributes one set of chromosomes, creating a diploid cell containing matched pairs of chromosomes called homologous (“same knowledge”) chromosomes. Homologous chromosomes are the same length and have specific nucleotide segments called genes in the same locus (location on the chromosome). Genes, the functional units of chromosomes, determine specific characteristics by coding for specific proteins. Different sequences of nucleotides at a particular gene, called alleles, code for different protein variations, resulting in variations in a trait. For example, the hair color that someone has is determined by which alleles they have in the genes that code for the proteins that determine hair color.
Each copy of a homologous pair of chromosomes originates from a different parent. Homologous chromosomes contain the same genes but may not be identical because they can contain different alleles. In other words, a particular locus may contain a gene for hair color, but the homologous chromosomes may have different alleles at that locus. The variation of individuals within a species is due to the specific combination of alleles inherited from both parents. Even a slightly altered sequence of nucleotides within a gene can result in an alternative trait. For example, three possible gene sequences on the human chromosome code for blood type: sequence A (called allele A), sequence B (called allele B), and sequence O (called allele O). Because all diploid human cells have two copies of the chromosome that determines blood type, the blood type (the trait) is determined by the two alleles of the gene that are inherited by in individual from their parents. It is possible to have two copies of the same gene sequence (allele) on both homologous chromosomes (for example, AA, BB, or OO) or two different sequences, such as AB, AO, or BO.
Variations of traits, such as blood type, eye color, and handedness, contribute to the natural variation found within a species. However, the difference is much less than 1% of the entire DNA sequence from any pair of human homologous chromosomes being compared. The sex chromosomes, X and Y, are the single exception to the rule of homologous chromosome uniformity: Other than a small amount of homology that is necessary to accurately produce gametes, the genes found on the X and Y chromosomes are different.
Meiosis is the nuclear division that forms gametes. In humans, typical body cells (somatic cells) are diploid, whereas gametes are haploid cells. Meiosis employs many of the same cellular mechanisms as mitosis. However, as you have learned, mitosis produces daughter cells whose nuclei are genetically identical to the original parent nucleus. In mitosis, the parent and the daughter nuclei have the same ploidy—diploid in the case of most animals. In meiosis, the resulting cells, or gametes, always have half the ploidy of the starting cells. To achieve this reduction in chromosome number, meiosis consists of one round of chromosome replication followed by two rounds of nuclear division. Because the events that occur during each division stage are analogous to the events of mitosis, the same stage names are assigned. However, because there are two division rounds, the major process and the stages are designated with an “I” or an “II.” Thus, meiosis I is the first round of meiotic division and consists of prophase I, prometaphase I, and so on. Likewise, meiosis II is the second round of meiotic division and consists of prophase II, prometaphase II, and so on.
Meiosis I
Meiosis is preceded by an interphase consisting of G1, S, and G2 phases, which are nearly identical to the phases preceding mitosis. The G1 phase (the “first gap phase”) is focused on cell growth. During the S phase, the cell replicates its DNA. Finally, in the G2 phase (the “second gap phase), the cell undergoes the final preparations for meiosis.
During DNA duplication in the S phase, each chromosome is replicated to produce two identical copies—sister chromatids that are held together at the centromere by cohesin proteins, which hold the chromatids together until anaphase II.
Prophase I
Early in prophase I, before the chromosomes can be seen clearly with a microscope, the homologous chromosomes are attached at their tips to the nuclear envelope by proteins. As the nuclear envelope begins to break down, the proteins associated with homologous chromosomes bring the pair closer together. Recall that in mitosis, homologous chromosomes do not pair together. The synaptonemal complex, a lattice of proteins between the homologous chromosomes, first forms at specific locations and then spreads outward to cover the entire length of the chromosomes. Homologous chromosomes pair up lengthwise in a process called synapsis. In synapsis, the genes on the chromatids of the homologous chromosomes are aligned precisely with each other. At this point the two homologous chromosomes are called a tetrad because there are a total of four chromatids, or two distinct sister chromatid pairs. The synaptonemal complex supports the exchange of chromosomal segments between homologous non-sister chromatids—a process called crossing over. Crossing over is the exchange of DNA segments between homologous chromosomes. It is an important source of genetic variation and will be discussed in depth in the later chapters. Crossing over can be observed visually after the exchange as chiasmata (Fig 7.3).
In humans, even though the X and Y sex chromosomes are not completely homologous (that is, most of their genes differ), a small region of homology allows the X and Y chromosomes to pair up during prophase I. A partial synaptonemal complex develops only between regions of homology.
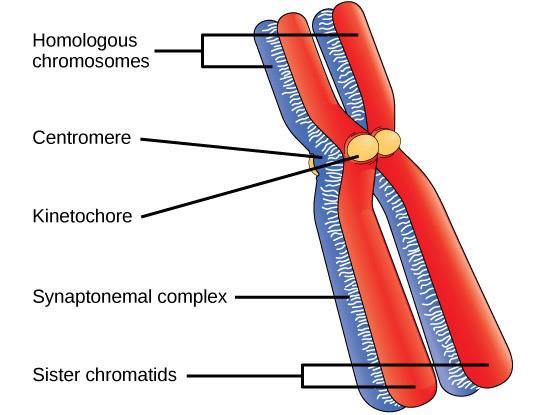
Prometaphase I
The key event in prometaphase I is the attachment of the spindle fiber microtubules to the kinetochore proteins at the centromeres. Kinetochore proteins are multiprotein complexes that bind the centromeres of a chromosome to the microtubules of the mitotic spindle. Microtubules grow from Microtubule-Organizing Centers (MTOCs). In animal cells, MTOCs are centrosomes located at opposite cell poles. The microtubules from each pole move toward the middle of the cell and attach to one of the kinetochores of the two fused homologous chromosomes. Each member of the homologous pair attaches to a microtubule extending from opposite poles of the cell so that in the next phase, the microtubules can pull the homologous pair apart. At the end of prometaphase I, each tetrad is attached to microtubules from both poles, with one homologous chromosome facing each pole. The homologous chromosomes are still held together at the chiasmata. In addition, the nuclear membrane has broken down entirely.
Metaphase I
During metaphase I, the homologous chromosomes are arranged at the metaphase plate—roughly in the cell’s midline, with the kinetochores facing opposite poles. The homologous pairs orient themselves randomly at the equator. For example, if the two homologous members of chromosome 1 are labeled a and b, then the chromosomes could line up a-b or b-a. This is important in determining the genes carried by a gamete, as each will only receive one of the two homologous chromosomes.
Anaphase I
In anaphase I, the microtubules pull the linked homologous chromosomes apart. The sister chromatids of each homologous chromosome remain tightly bound together at the centromere. The chiasmata are broken in anaphase I as the microtubules attached to the fused kinetochores pull the homologous chromosomes apart.
Telophase I and Cytokinesis
In telophase, the separated chromosomes arrive at opposite poles. Depending on the species, the remainder of the typical telophase events may or may not occur. In some organisms, the chromosomes decondense and nuclear envelopes form around the separated sets of chromatids produced during telophase I. In other organisms, cytokinesis—the physical separation of the cytoplasmic components into two daughter cells— occurs without the reformation of the nuclei. In nearly all species of animals and some fungi, cytokinesis separates the cell contents via a cleavage furrow (constriction of the actin ring that leads to cytoplasmic division). In plants, a cell plate is formed during cell cytokinesis by Golgi vesicles fusing at the metaphase plate. This cell plate will ultimately lead to the formation of cell walls that separate the two daughter cells.
Meiosis I product
Two haploid cells result from the first meiotic division of a diploid cell. The cells are haploid because, at each pole, there is just one of each pair of homologous chromosomes. Therefore, only one complete set of chromosomes is present. Thus, the resulting cells are considered haploid because there is only one chromosome set, even though each chromosome still consists of two sister chromatids. Recall that sister chromatid are duplicates of one of the two homologous chromosomes (except for changes that occurred during crossing over). We will see in the next chapter that in meiosis II, these two sister chromatids will separate, creating four haploid daughter cells.
Reading Check #3
What is the result of the first meiotic division in terms of ploidy?
A. Diploid cells
B. Triploid cells
C. Tetraploid cells
D. Haploid cells
Reading Question #4
Which process separates the two homologous chromosomes and creates two haploid daughter cells?
A. Mitosis
B. Mieosis I
C. Meiosis II
D. Cytokinesis II
Reading Question #5
In a certain species, a diploid parent cell has 12 chromosomes. How many chromosomes will each haploid daughter cell have after the completion of meiosis?
A. 6 chromosomes
B. 12 chromosomes
C. 24 chromosomes
D. 48 chromosomes
References and Acknowledgements
University of Washington. (2021, August 11). New study analyzes role of scent compounds in the coevolution of bats and pepper plants. ScienceDaily. Retrieved from www.sciencedaily.com/releases/2021/08/210811162901.htm
Van Valen, L. (1973). “A New Evolutionary Law,” Evolutionary Theory 1(1):30 doi: 10.7208/9780226115504-022
Adapted from Clark, M.A., Douglas, M., and Choi, J. (2018, March 28). Biology 2e. OpenStax. Retrieved from https://openstax.org/books/biology-2e/pages/1-introduction