6 Chapter 6: Eukaryotic DNA Replication and Repair
Joshua Reid; Lisa Limeri; and rocksher
Learning Objectives
By the end of this section, you should be able to:
- Explain 1) the problem that arises during lagging strand synthesis at the end of a chromosome, and 2) the role of telomerase in solving the problem.
- Explain how DNA damage and/or mismatches are detected and repaired.
- Given a specific type of DNA mutation or damage, predict which DNA repair pathway would respond, then predict the consequences of successful and unsuccessful repair.
DNA Replication in Eukaryotes
Eukaryotic genomes are much more complex and larger in size than prokaryotic genomes. Eukaryotes also have several different linear chromosomes, as opposed to a single circular chromosome. The linear structure of the eukaryotic chromosomes introduces unique challenges to DNA replication
Telomere Replication
Unlike prokaryotic chromosomes, eukaryotic chromosomes are linear. As you’ve learned, the enzyme DNA pol can add nucleotides only in the 5′ to 3′ direction. On the leading strand, synthesis continues until the end of the chromosome is reached. On the lagging strand, DNA is synthesized in short stretches, each of which is initiated by a separate primer. When the replication fork reaches the end of the linear chromosome, there is no way to replace the primer on the 5′ end of the lagging strand. The DNA at the ends of the chromosome thus remains unpaired, and over time these ends, called telomeres, may get progressively shorter as cells continue to divide.
Telomeres comprise repetitive sequences that code for no particular gene. In humans, a six-base-pair sequence, TTAGGG, is repeated 100 to 1000 times in the telomere regions. In a way, these telomeres protect the genes from getting deleted as cells continue to divide. The telomeres are added to the ends of chromosomes by a separate enzyme, telomerase (Fig 6.1), whose discovery helped in the understanding of how these repetitive chromosome ends are maintained. The telomerase enzyme contains a catalytic part and a built-in RNA template. It attaches to the end of the chromosome, and DNA nucleotides complementary to the RNA template are added on the 3′ end of the DNA strand. Once the 3′ end of the lagging strand template is sufficiently elongated, DNA polymerase can add the nucleotides complementary to the ends of the chromosomes. Thus, the ends of the chromosomes are replicated.
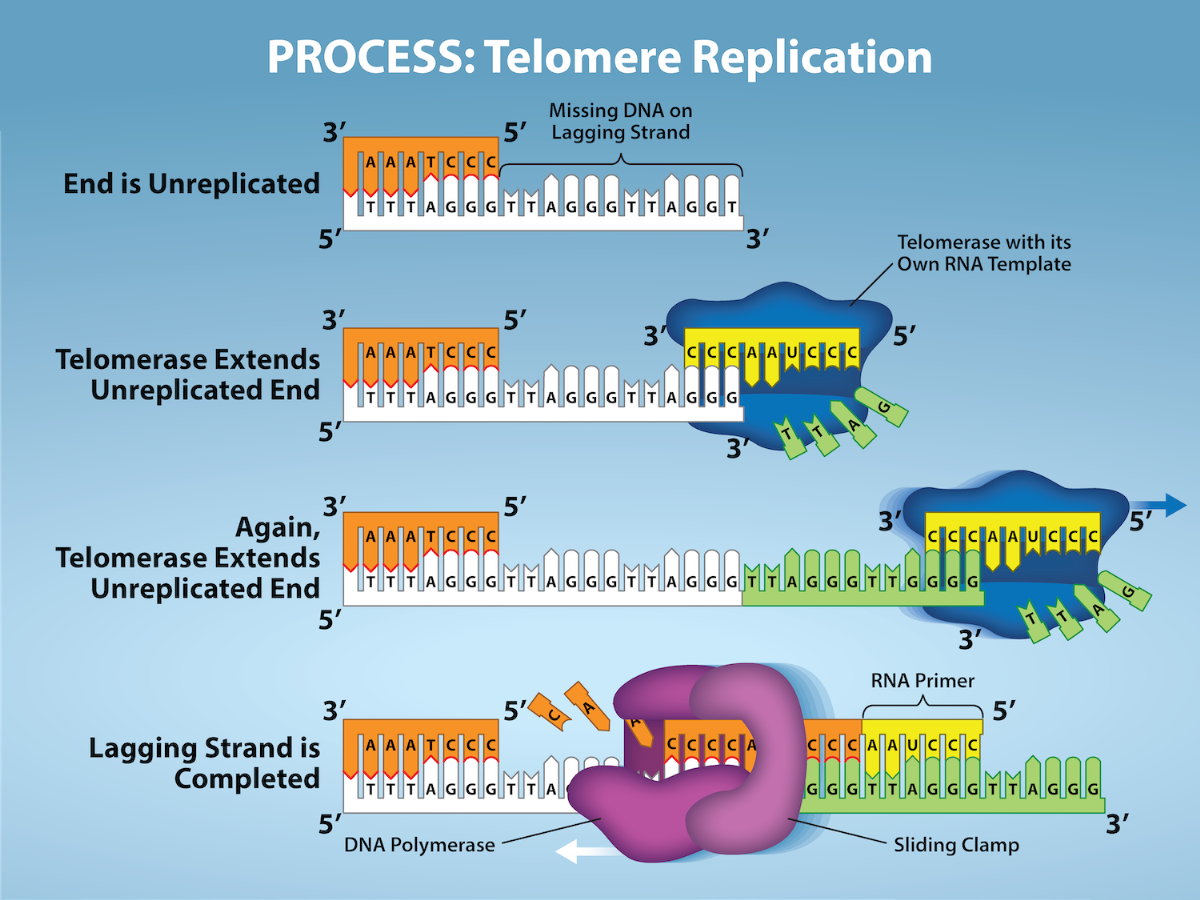
Telomerase is typically active in germ cells and adult stem cells. It is not active in adult somatic cells. For their discovery of telomerase and its action, Elizabeth Blackburn, Carol W. Greider, and Jack W. Szostak (Figure 6.2) received the Nobel Prize for Medicine and Physiology in 2009. Later research using HeLa cells (obtained from Henrietta Lacks) confirmed that telomerase is present in human cells. And in 2001, researchers, including Diane L. Wright, found that telomerase is necessary for cells in human embryos to rapidly proliferate.
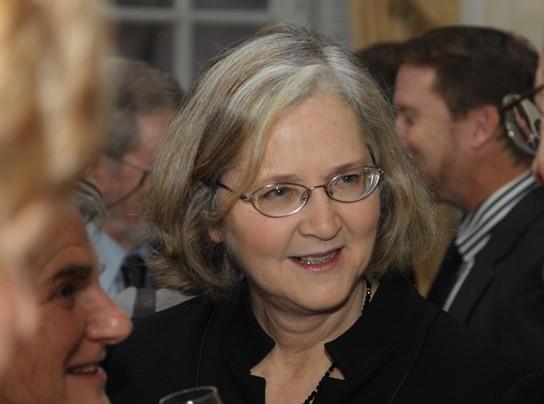
Telomerase and Aging
Cells that undergo cell division continue to have their telomeres shortened because most somatic cells do not make telomerase. This essentially means that telomere shortening is associated with aging.
In 2010, scientists found that telomerase can reverse some age-related conditions in mice. This may have potential in regenerative medicine (Jaskelioff et al., 2011) Telomerase-deficient mice were used in these studies; these mice have tissue atrophy, stem cell depletion, organ system failure, and impaired tissue injury responses. Telomerase reactivation in these mice caused the extension of telomeres, reduced DNA damage, reversed neurodegeneration, and improved the function of the testes, spleen, and intestines.
Cancer is characterized by uncontrolled cell division of abnormal cells. The cells accumulate mutations, proliferate uncontrollably, and can migrate to different parts of the body through a process called metastasis. Scientists have observed that cancerous cells have considerably shortened telomeres and that telomerase is active in these cells. Interestingly, only after the telomeres were shortened in the cancer cells did the telomerase become active. If the action of telomerase in these cells can be inhibited by drugs during cancer therapy, then the cancerous cells could potentially be stopped from further division.
Reading Check #1
Which of the following is a function of telomeres?
A. To help make Okazaki fragments.
B. To protect DNA polymerase.
C. To protect the ends of chromosomes.
D. To prevent cancer.
Reading Check #2
Which enzyme is responsible for adding nucleotides to the 3′ end of the DNA strand during telomere replication in eukaryotes?
A. DNA Polymerase I
B. Primase
C. Telomerase
D. DNA Polymerase ɑ
DNA Repair Mechanisms
DNA replication is a highly accurate process, but mistakes can occasionally occur, such as a DNA polymerase inserting a wrong base. Uncorrected mistakes may sometimes lead to serious consequences, such as cancer. Repair mechanisms correct the mistakes. In rare cases, mistakes are not corrected, leading to mutations; in other cases, repair enzymes are themselves mutated or defective.
Most of the mistakes during DNA replication are promptly corrected by the proofreading ability of DNA polymerase itself (Fig 6.3). In proofreading, the DNA pol reads the newly added base before adding the next one, so a correction can be made. The polymerase checks whether the newly added base has paired correctly with the base in the template strand. If it is the right base, the next nucleotide is added. If an incorrect base has been added, the enzyme makes a cut at the phosphodiester bond and releases the wrong nucleotide. This is performed by the 3′ exonuclease action of DNA pol. Once the incorrect nucleotide has been removed, it can be replaced by the correct one.
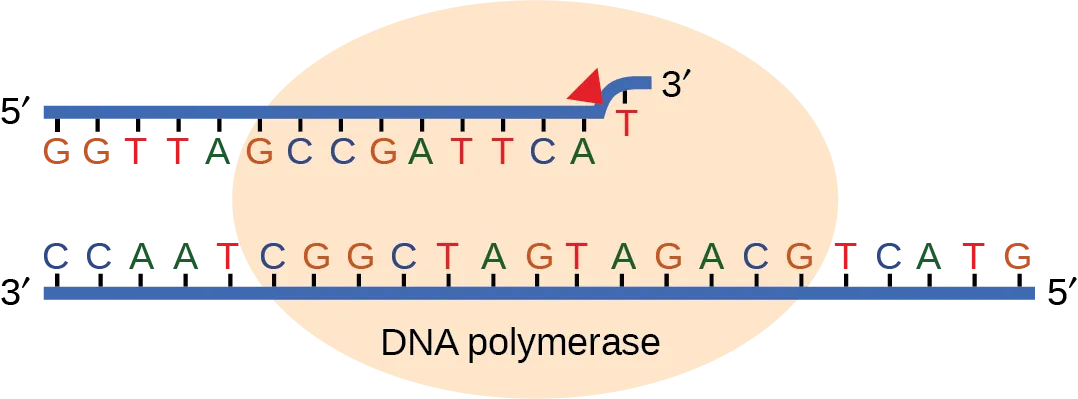
Some errors are not corrected during replication, but are instead corrected after replication is completed; this type of repair is known as mismatch repair (Fig. 6.4). Specific repair enzymes recognize the mispaired nucleotide and excise part of the strand that contains it; the excised region is then resynthesized. If the mismatch remains uncorrected, it may lead to more permanent damage when the mismatched DNA is replicated. How do mismatch repair enzymes recognize which of the two bases is the incorrect one? In E. coli, after replication, the nitrogenous base adenine acquires a methyl group; the parental DNA strand will have methyl groups, whereas the newly synthesized strand lacks them. Thus, DNA polymerase is able to remove the wrongly incorporated bases from the newly synthesized, non-methylated strand. In eukaryotes, the mechanism is not very well understood, but it is believed to involve recognition of unsealed nicks in the new strand, as well as a short-term continuing association of some of the replication proteins with the new daughter strand after replication has completed.
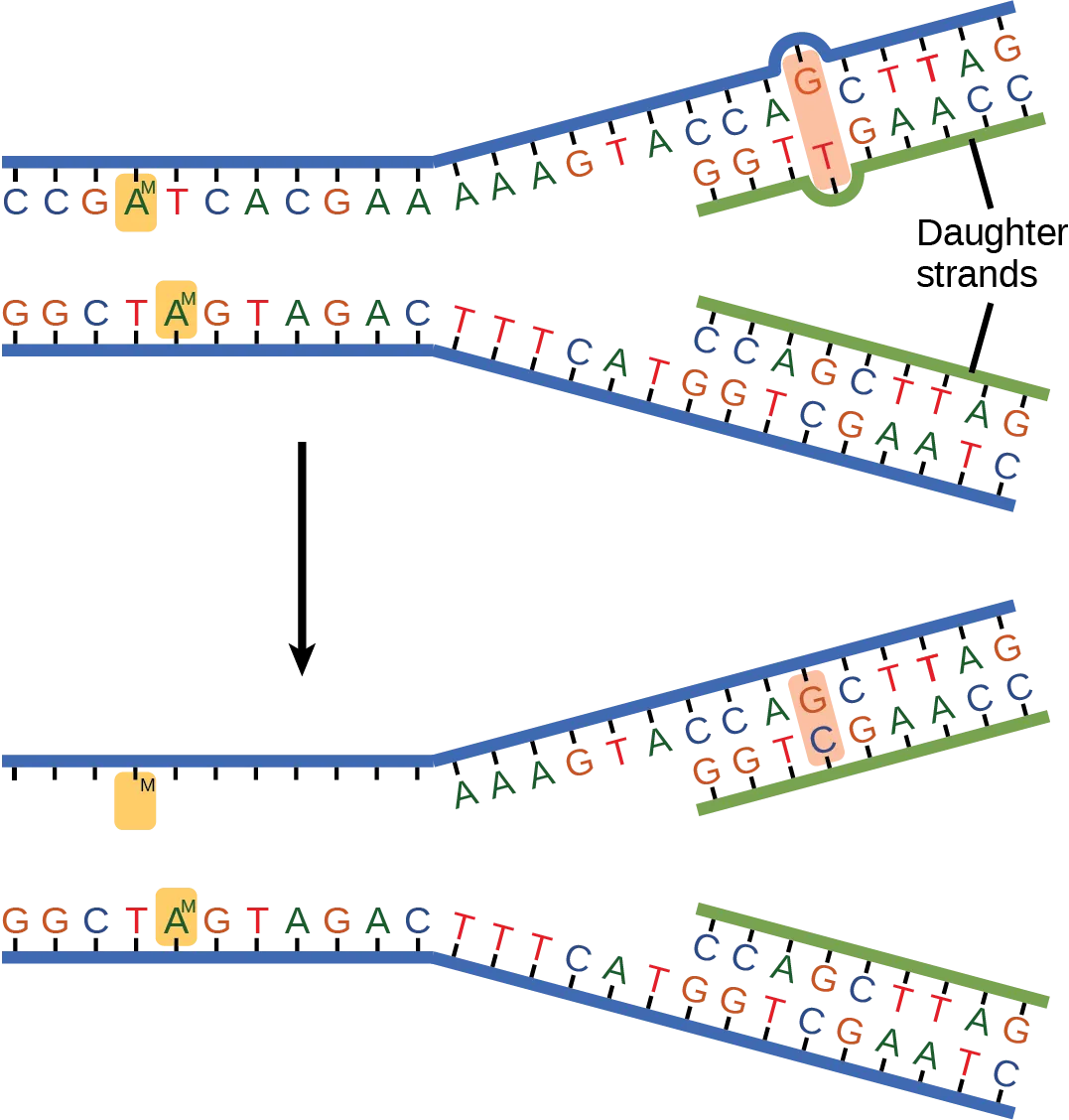
Another type of repair mechanism, nucleotide excision repair, is similar to mismatch repair, except that it is used to remove damaged bases rather than mismatched ones. The repair enzymes replace abnormal bases by making a cut on both the 3′ and 5′ ends of the damaged base (Fig 6.5). The segment of DNA is removed and replaced with the correctly paired nucleotides by the action of DNA pol. Once the bases are filled in, the remaining gap is sealed with a phosphodiester linkage catalyzed by DNA ligase. This repair mechanism is often employed when UV exposure causes the formation of pyrimidine dimers.
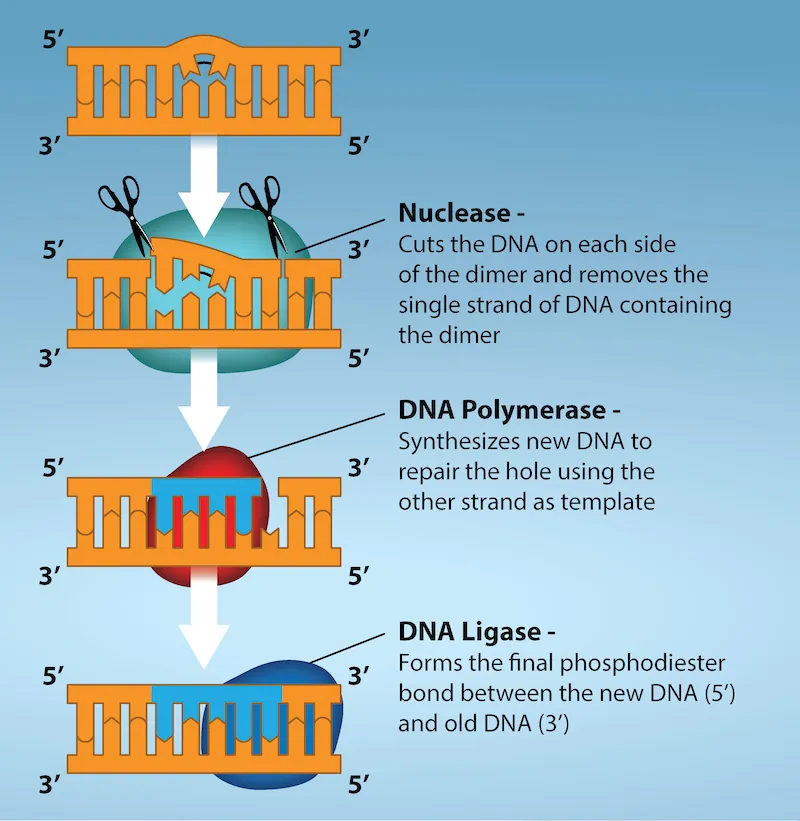
A well-studied example of mistakes not being corrected is seen in people suffering from xeroderma pigmentosa (Fig 6.6). Affected individuals have skin that is highly sensitive to UV rays from the sun. When individuals are exposed to UV light, pyrimidine dimers, especially those of thymine, are formed; people with xeroderma pigmentosa are not able to repair the damage. These are not repaired because of a defect in the nucleotide excision repair enzymes, whereas in normal individuals, the thymine dimers are excised and the defect is corrected. The thymine dimers distort the structure of the DNA double helix, and this may cause problems during DNA replication. People with xeroderma pigmentosa may have a higher risk of contracting skin cancer than those who don’t have the condition.
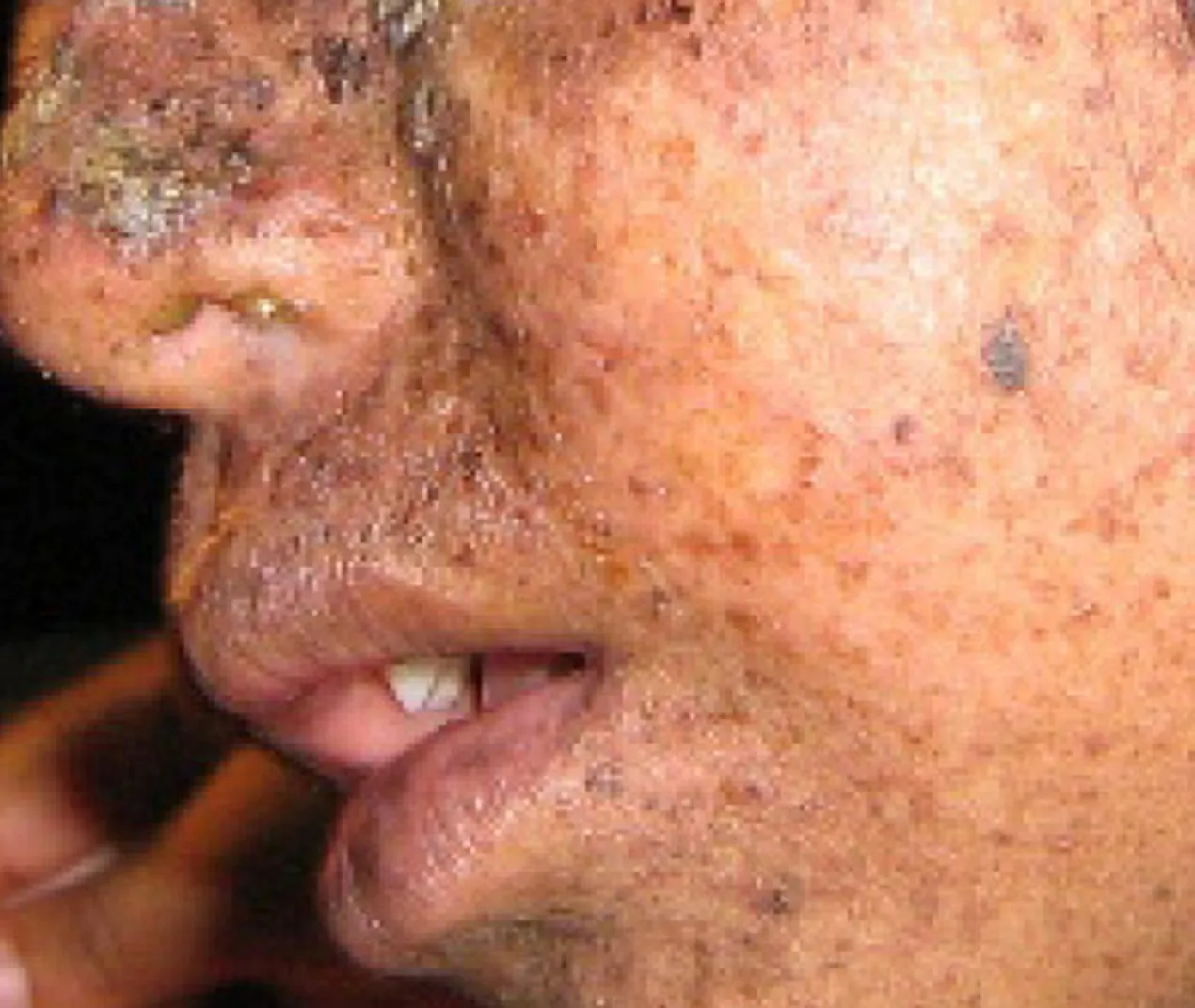
Errors during DNA replication are not the only reason why mutations arise in DNA. Mutations, variations in the nucleotide sequence of a genome, can also occur because of damage to DNA. Such mutations may be of two types: induced or spontaneous. Induced mutations are those that result from an exposure to chemicals, UV rays, x-rays, or some other environmental agent. For example, Charlotte Auerbach and J.M Robson discovered the mutation-inducing effects of mustard gas. Spontaneous mutations occur without any exposure to any environmental agent; they are a result of natural reactions taking place within the body.
Mutations may have a wide range of effects. Point mutations are those mutations that affect a single base pair. The most common nucleotide mutations are substitutions, in which one base is replaced by another. These substitutions can be of two types, either transitions or transversions. Transition substitution refers to a purine or pyrimidine being replaced by a base of the same kind; for example, a purine such as adenine may be replaced by the purine guanine. Transversion substitution refers to a purine being replaced by a pyrimidine, or vice versa; for example, cytosine, a pyrimidine, is replaced by adenine, a purine. Some point mutations are not detectable in the final product; these are known as silent mutations. Silent mutations are usually due to a substitution in the third base of a codon, which often represents the same amino acid as the original codon. Other point mutations can result in the replacement of one amino acid by another, which may alter the function of the protein. Point mutations that generate a stop codon can terminate a protein early.
Some mutations can result in an increased number of copies of the same codon. These are called trinucleotide repeat expansions and result in repeated regions of the same amino acid. Mutations can also be the result of the addition of a base, known as an insertion, or the removal of a base, also known as deletion. If an insertion or deletion results in the alteration of the translational reading frame (a frameshift mutation), the resultant protein is usually nonfunctional. Sometimes a piece of DNA from one chromosome may get translocated to another chromosome or to another region of the same chromosome; this is also known as translocation. These mutations are visualized in figure 6.7.
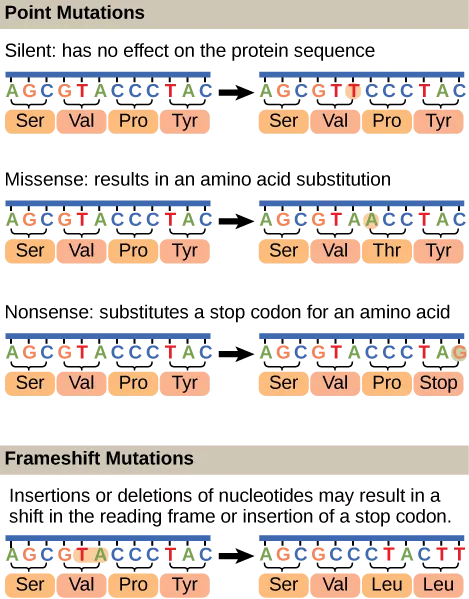
Reading Check #3
Which of the following is NOT a DNA repair mechanism?
A. Mismatch repair
B. Nucleotide excision repair
C. Binding-protein excision repair
D. Base excision pair
Reading Check #4
Which type of mutation involves the replacement of one base with another, potentially altering the protein’s function?
A. Silent mutation
B. Transition substitution
C. Deletion
D. Frameshift mutation
Reading Check #5
In eukaryotes, the mismatch repair mechanism is initiated and directed by
A. Methylated DNA stand
B. Acetylated DNA strand
C. Strand specific nicks
D. Double stranded breaks
Mutations in repair genes have been known to cause cancer. Many mutated repair genes have been implicated in certain forms of pancreatic cancer, colon cancer, and colorectal cancer. Mutations can affect either somatic cells or germ cells. If many mutations accumulate in a somatic cell, they may lead to problems such as the uncontrolled cell division observed in cancer. If a mutation takes place in germ cells, the mutation will be passed on to the next generation, as in the case of hemophilia and xeroderma pigmentosa.
Acknowledgements
Adapted from Clark, M.A., Douglas, M., and Choi, J. (2018). Biology 2e. OpenStax. Retrieved from https://openstax.org/books/biology-2e/pages/1-introduction