23 Chapter 23: Photosynthesis: Light-dependent Reactions
Lisa Limeri
Learning Objectives
By the end of this section, students will be able to:
- In photosynthesis reactions, identify the atoms that lose electrons, the atoms that gain electrons, the molecules that are reduced, and the molecules that are oxidized. Explain the impacts of these changes on the energy available in each molecule.
- Create a model that describes how light energy is transformed into chemical energy in the light-dependent reactions in C3 photosynthesis. Key terms your model should include: chloroplast, thylakoid, stroma, pigments, PSII, PSI, H20, O2, electrons, sunlight, H+, ATP synthase, ADP/ATP, NADP+/NADPH, reduction, and oxidation.
- Describe the main cellular structures, and their functions, required for C3 photosynthesis: chloroplast, thylakoid, granum, stroma, pigments.
Introduction
The metabolic processes in all organisms—from bacteria to humans—require energy. To get this energy, many organisms access stored energy by ingesting other organisms. But where does the stored energy in food originate? All of this energy can be traced back to photosynthesis.
Photosynthesis is essential to all life on earth; both plants and animals depend on it. It is the only biological process that can capture energy that originates from sunlight and converts it into chemical compounds (carbohydrates) that many organisms use to power their metabolism. It is also a source of oxygen necessary for many living organisms. How can light energy be used to make food? When a person turns on a lamp, electrical energy becomes light energy. Like all other forms of kinetic energy, light can travel, change form, and be harnessed to do work. In the case of photosynthesis, light energy is converted into chemical energy.
Energy in Living Systems
Energy production within a cell involves many coordinated chemical pathways. Most of these pathways are combinations of oxidation and reduction reactions, which occur at the same time. An oxidation reaction strips an electron from an atom in a compound, and the addition of this electron to another compound is a reduction reaction. Because oxidation and reduction occur together (an electron being taken from somewhere must be given somewhere else), these pairs of reactions are called reduction-oxidation reactions, or redox reactions (Fig 23.1).
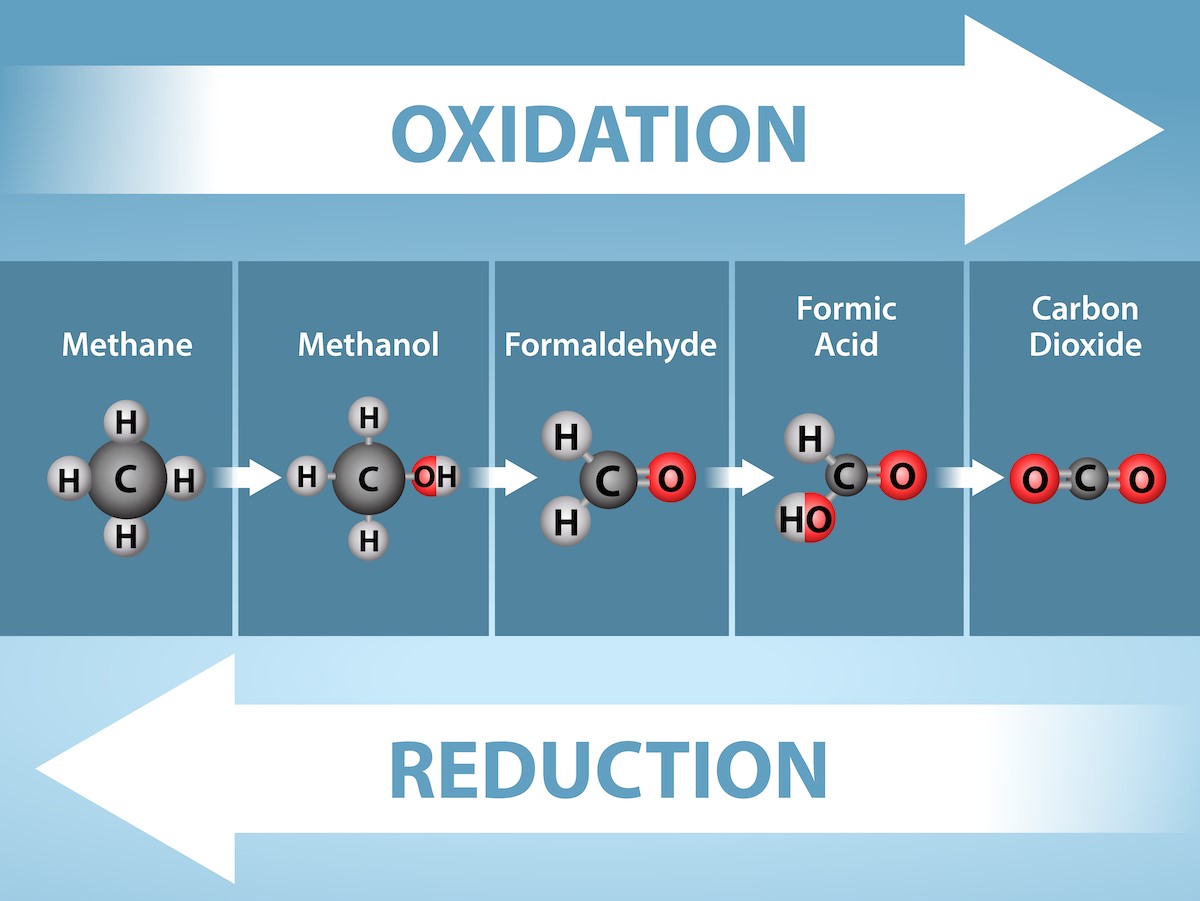
Electrons and Energy
Removing electrons from a molecule (oxidation) results in a decrease in potential energy in the oxidized compound. The electron does not remain unbonded; it is shifted to a second compound, reducing the second compound. The shift of an electron from one compound to another moves some potential energy from the first compound (the oxidized compound) to the second compound (the reduced compound). Thus, in redox reactions, the oxidized compound loses some potential energy and the reduced compound gains some potential energy.
The transfer of electrons between molecules is important because most of the energy stored in atoms and used to fuel cell functions is in the form of high-energy electrons. The transfer of energy in the form of high energy electrons allows the cell to transfer and use energy in an incremental fashion—in small packages rather than in a single, destructive burst. This module focuses on the conversion of light energy to chemical energy in the light-dependent reactions of photosynthesis; you will see that as you track the path of the transfers, you are tracking the path of electrons moving through metabolic pathways.
Electron Carriers
In living systems, a small class of compounds functions as electron shuttles: they bind and carry high-energy electrons between compounds in biochemical pathways. The principal electron carriers we will consider are derived from the B vitamin group and are derivatives of nucleotides. These compounds can be easily reduced (that is, they accept electrons) or oxidized (they lose electrons). Nicotinamide adenine dinucleotide phosphate (NADP+) (Figure 23.2) is derived from vitamin B3, niacin. NADP+ is the oxidized form of the molecule; NADPH is the reduced form of the molecule after it has accepted two electrons and a proton (which together are the equivalent of a hydrogen atom with an extra electron; Fig 23.2). Note that if a compound has an “H” on it, it is generally reduced (e.g., NADPH is the reduced form of NADP+).
When electrons are added to a compound, it is reduced. A compound that reduces another is called a reducing agent. When electrons are removed from a compound, it is oxidized. A compound that oxidizes another is called an oxidizing agent. NADP+ plays an important role in photosynthesis. Similarly, flavin adenine dinucleotide (FAD) is derived from vitamin B2, also called riboflavin. Its reduced form is FADH2. A second variation of NADP, called NAD+, also acts as an oxidizing agent, capturing high energy electrons to form NADH during different stages of carbohydrate catabolism in cellular respiration.
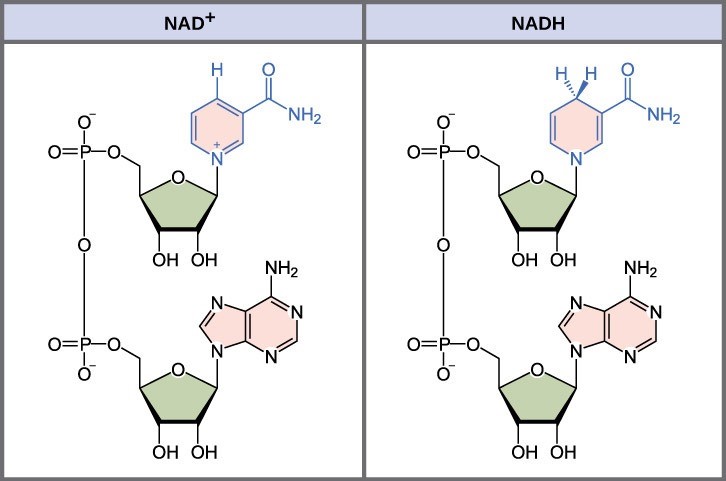
Reading Question #1
When a molecule gains an electron, it…
A. is reduced and gains energy.
B. is reduced and loses energy.
C. is oxidized and gains energy.
D. is oxidized and loses energy.
Overview of Photosynthesis
During photosynthesis, the energy of sunlight is “captured” to energize electrons, whose energy is then stored in the covalent bonds of sugar molecules. How long lasting and stable are those covalent bonds? The energy extracted today by the burning of coal and petroleum products represents sunlight energy captured and stored by photosynthesis 350 to 200 million years ago during the Carboniferous Period. Plants, green algae, and a group of bacteria called cyanobacteria are the only organisms capable of performing photosynthesis (Figure 23.3).

The importance of photosynthesis is not just that it can capture sunlight’s energy. After all, a lizard sunning itself on a cold day can use the sun’s energy to warm up. In contrast, photosynthesis is vital because it evolved as a way to store the energy from solar radiation (the “photo-” part) to energy in the carbon-carbon bonds of carbohydrate molecules (the “-synthesis” part). Those carbohydrates are the energy source that other organisms use to power the synthesis of ATP via cellular respiration, which we will learn about in more detail in the next unit. Therefore, photosynthesis powers 99% of Earth’s ecosystems. When a top predator, such as a wolf, preys on a deer, the wolf is at the end of an energy path that went from nuclear reactions on the surface of the sun, to visible light, to photosynthesis, to vegetation, to deer, and finally to the wolf.
Photosynthesis is a multi-step process that requires specific wavelengths of visible sunlight, carbon dioxide (which is low in energy), and water as substrates (Figure 23.4). After the process is complete, it releases oxygen and produces glyceraldehyde-3-phosphate (G3P), which are simple carbohydrate molecules that are high in energy and can be converted into glucose, sucrose, or any of dozens of other sugar molecules.
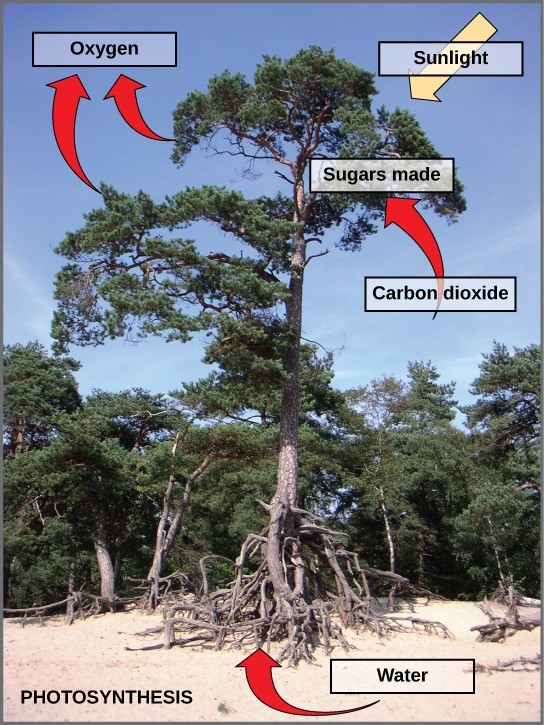
The following is the chemical equation for photosynthesis (Figure 23.5):
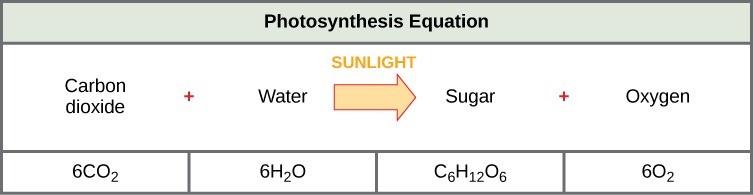
Photosynthesis takes place in two sequential stages: first the light-dependent reactions and then light-independent reactions (Fig. 23.6). In the light-dependent reactions, energy from sunlight is absorbed by chlorophyll and that energy is converted into stored chemical energy. In the light-independent reactions, the chemical energy harvested during the light-dependent reactions drives the assembly of sugar molecules from carbon dioxide.
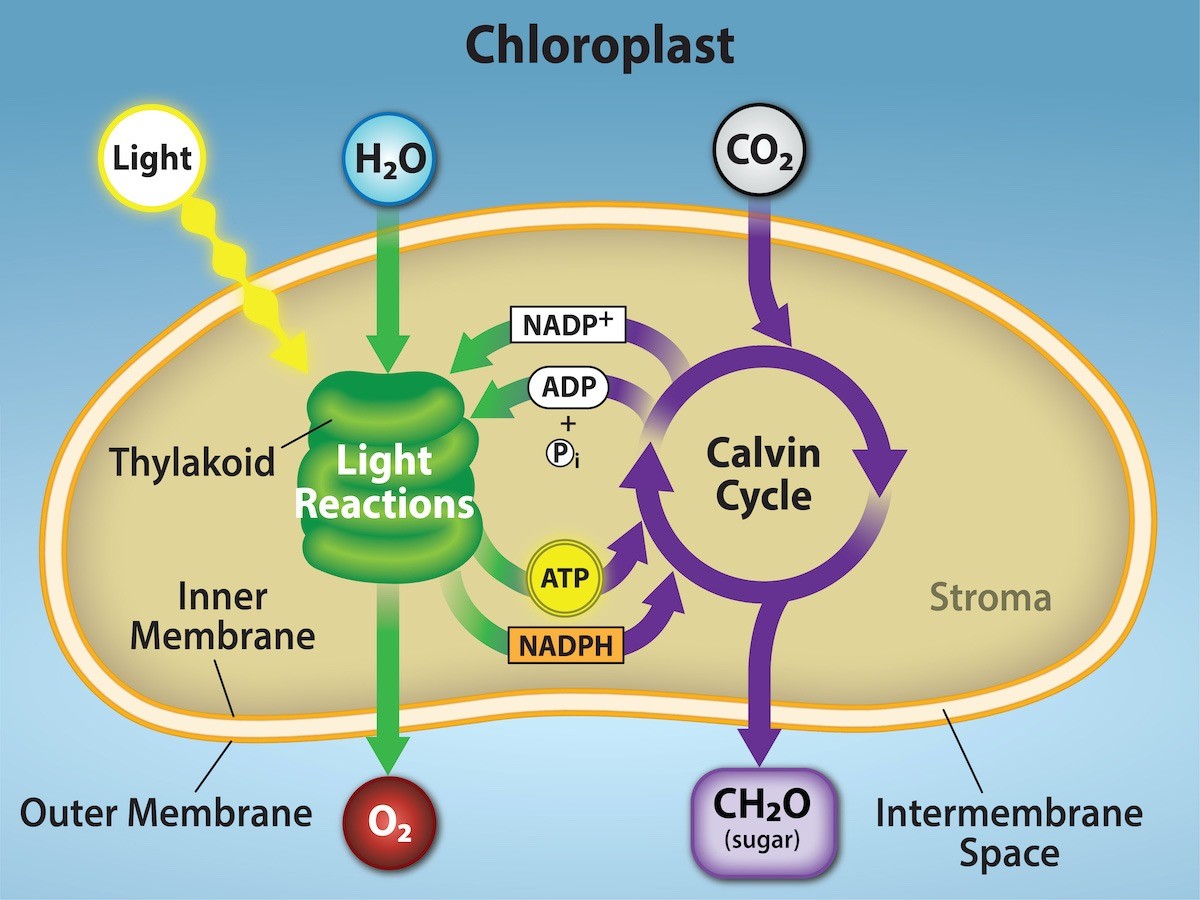
Everyday Connection: Photosynthesis at the Grocery Store
Major grocery stores in the United States are organized into departments, such as dairy, meats, produce, bread, cereals, and so forth. Each aisle (Figure 26.10) contains hundreds, if not thousands, of different products for customers to buy and consume.
Although there is a large variety, each item ultimately can be linked back to photosynthesis. Meats and dairy link, because the animals were fed plant-based foods. The breads, cereals, and pastas come largely from starchy grains, which are the seeds of photosynthesis-dependent plants. What about desserts and drinks? All of these products contain sugar— sucrose is a plant product, a disaccharide, a carbohydrate molecule, which is built directly from photosynthesis. Moreover, many items are less obviously derived from plants: For instance, paper goods are generally plant products, and many plastics (abundant as products and packaging) are derived from “algae” (unicellular plant-like organisms, and cyanobacteria). Virtually every spice and flavoring in the spice aisle was produced by a plant as a leaf, root, bark, flower, fruit, or stem. Ultimately, photosynthesis connects to every meal and every food a person consumes.
Reading Question #2
During photosynthesis, energy is converted from ___ energy into ___ energy.
A. light; chemical
B. chemical; chemical
C. mechanical; light
D. kinetic; chemical
Photosynthesis in Plants Takes Place in Chloroplasts
In plants, photosynthesis generally takes place in leaves, which consist of several layers of cells. The process of photosynthesis occurs in a middle layer called the mesophyll. The gas exchange of carbon dioxide and oxygen occurs through small, regulated openings called stomata (singular: stoma), which also play roles in the regulation of gas exchange and water balance (Fig 23.7). The stomata are typically located on the underside of the leaf, which helps to minimize water loss due to high temperatures on the upper surface of the leaf. Each stoma is flanked by guard cells that regulate the opening and closing of the stomata by swelling or shrinking in response to osmotic changes (Fig 23.7).
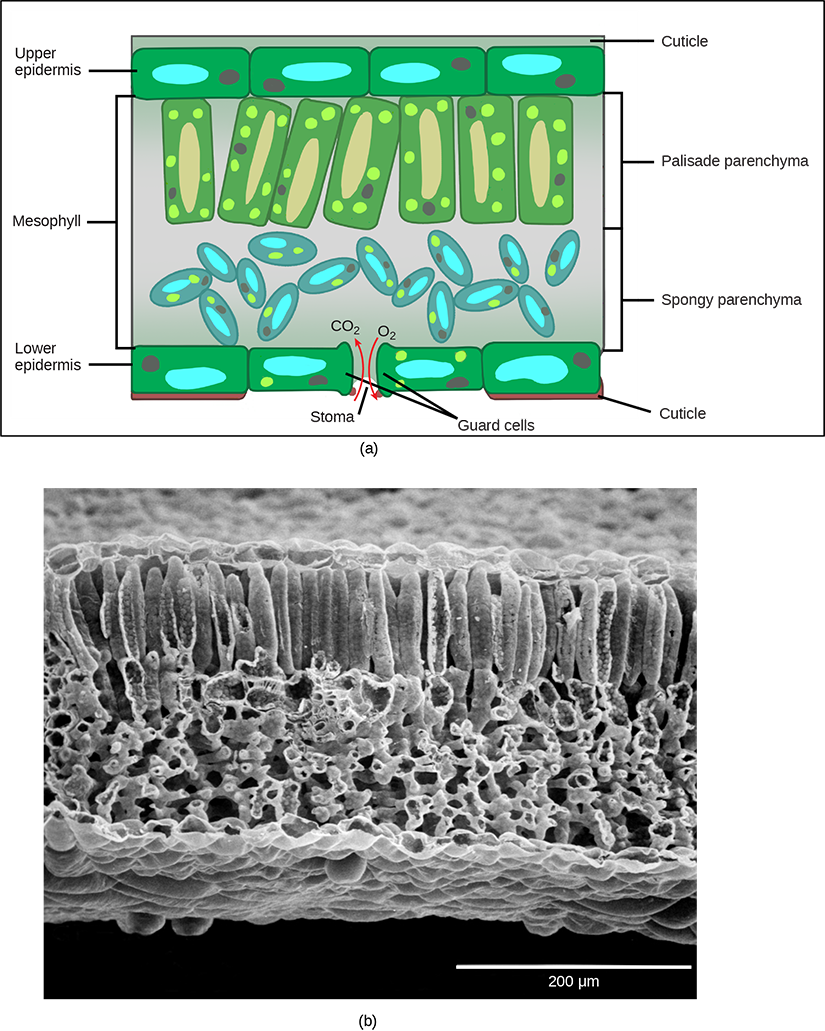
In plant cells, photosynthesis takes place inside an organelle called a chloroplast (Fig. 23.8). For plants, chloroplast-containing cells exist mostly in the mesophyll. Chloroplasts have a double membrane envelope (composed of an outer membrane and an inner membrane) and are ancestrally derived from ancient free-living cyanobacteria. Within the chloroplast are stacked, disc-shaped structures called thylakoids (Fig. 23.8). Embedded in the thylakoid membrane is chlorophyll, a pigment (molecule that absorbs light) responsible for the initial interaction between light and plant material, and numerous proteins that make up the electron transport chain. The thylakoid membrane encloses an internal space called the thylakoid lumen. A stack of thylakoids is called a granum, and the liquid-filled space surrounding the granum is called stroma (not to be confused with stoma, an opening on the leaf epidermis) (Fig. 23.8).
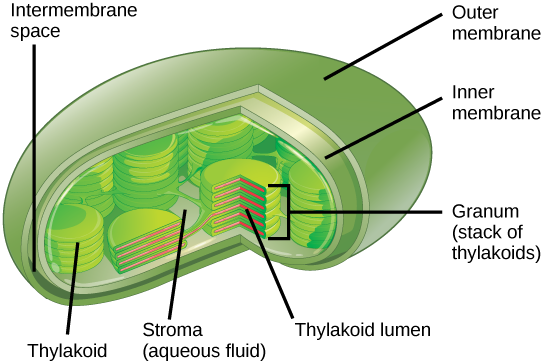
Reading Question #3
Where are the light-capturing pigments (such as chlorophyll) located?
A. Mitochondria
B. Nucleus
C. Thylakoid lumen
D. Thylakoid membrane
Research Connection: Marie Clark Taylor
As we have learned in this chapter, light is an important component of a plant’s environment. Understanding the relationship between light and plant development is what allows gardeners to select certain plants for certain garden conditions.
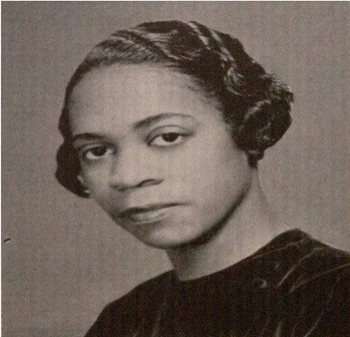
Dr. Marie Clark Taylor was the first woman to earn a scientific doctorate degree from Fordham University, and the first African-American woman to earn a doctorate degree in botany (Pizza, 2021). Her dissertation”examined the effect that light photoperiods (periods of daily light) have on the development of the cells that give rise to flowers” (NYBG, 2022). Dr. Taylor exposed different plants (scarlet sage, cosmos, and orange cosmos) to different periods of daily light (6, 10, or 16 hours) and recorded their seed and flower production. With 6 hours of light, scarlet sage produced flowers slower than normal, and with 16 hours of light, they did not produce flowers at all. The cosmos plants produced more flowers with longer periods of light, but the quality of flowers decreased with 16 hours of light (NYBG, 2022; Pizza, 2020). Plants have adapted to thrive under particular conditions. For example, plants that commonly grow in the shade have adapted to low levels of light by changing the relative concentrations of their chlorophyll pigments.
The light-dependent reactions of photosynthesis
The overall function of light-dependent reactions is to convert solar energy into chemical energy in the form of NADPH and ATP. This chemical energy supports the light-independent reactions and fuels the assembly of sugar molecules, which we will learn more about in the next chapter. Protein complexes and pigment molecules work together to produce NADPH and ATP.
Absorption of Light Energy
Light energy of specific wavelengths is absorbed by pigments. Chlorophylls and carotenoids are the two major classes of photosynthetic pigments found in plants and algae; each class has multiple types of pigment molecules. Chlorophyll a and chlorophyll b are found in plants. With dozens of different forms, carotenoids are a much larger group of pigments. The carotenoids found in fruit—such as the red of tomato (lycopene), the yellow of corn seeds (zeaxanthin), or the orange of an orange peel (β-carotene)—are used as advertisements to attract seed dispersers. In photosynthesis, carotenoids function as photosynthetic pigments that are very efficient molecules for the disposal of excess energy. When a leaf is exposed to full sun, the light-dependent reactions are required to process an enormous amount of energy; if that energy is not handled properly, it can do significant damage. Therefore, many carotenoids reside in the thylakoid membrane, absorb excess energy, and safely dissipate that energy as heat.
Many photosynthetic organisms have a mixture of pigments, and by using these pigments, the organism can absorb energy from a wider range of wavelengths. When a photon of light hits a pigment molecule, we say it is “absorbed.” What this means is that the energy from the photon excites an electron, moving it to a higher energy orbital (Figure 23.10).
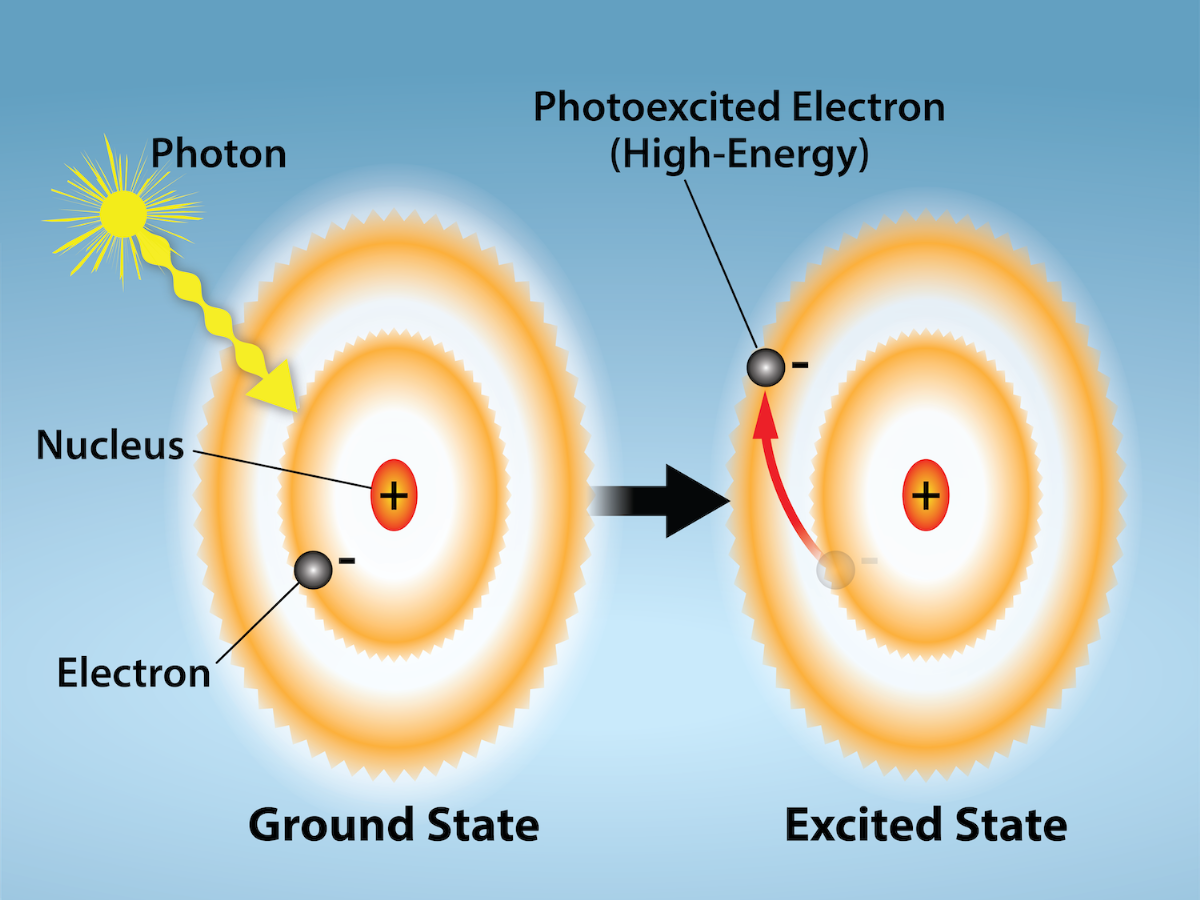
Photosystems
The actual step that converts light energy into chemical energy takes place in a multiprotein complex called a photosystem, two types of which are found embedded in the thylakoid membrane: photosystem II (PSII) and photosystem I (PSI) (Figure 23.11). The two complexes differ on the basis of what they oxidize (that is, the source of the low-energy electron supply) and what they reduce (the place to which they deliver their energized electrons). The numbering of the photosystems is derived from the order in which they were discovered, which happens to be the opposite of the order of the transfer of electrons.
Both photosystems have the same basic structure; a number of antenna proteins, which bind to the chlorophyll and other pigment molecules. The antenna proteins and pigments make up the light-harvesting complex, which surrounds the reaction center where the photochemistry takes place. The antenna proteins and pigments of the light harvesting complex function to pass energy from sunlight to the reaction center. A photon is absorbed by a pigment molecule (usually chlorophyll) and “excites” electrons by elevating them to a higher-energy shell (Figure 23.10). When this happens, the light energy has now been captured by biological molecules but is not stored in any useful form yet. The electron shortly after falls back to its resting state, releasing energy that excited an electron in the orbital shell of another nearby molecule in the antenna proteins. This process continues until eventually (after about a millionth of a second), it is delivered to the reaction center (Figure 23.11). Up to this point, only energy has been transferred between molecules, not electrons.
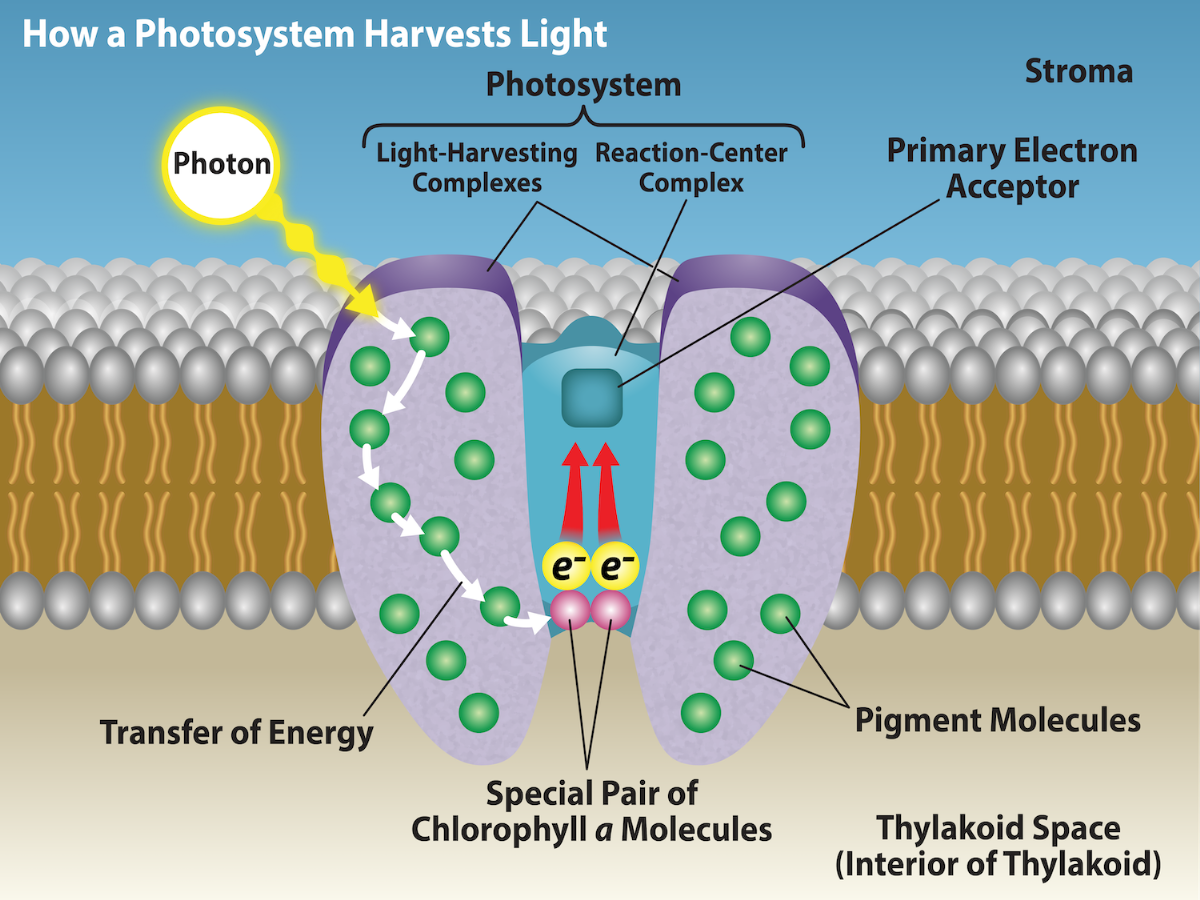
The reaction center contains a pair of chlorophyll a molecules with a special property. Those two chlorophylls can undergo oxidation upon excitation, meaning they can actually give up an electron. It is at this step in the reaction center during photosynthesis that light energy is converted into an excited electron. In the photosystem II (PSII) reaction center, energy from sunlight is used to extract electrons from water (Figure 23.12). These electrons are passed to the electron transport chain to photosystem I (PSI), which reduces NADP+ to NADPH (Figure 23.12).
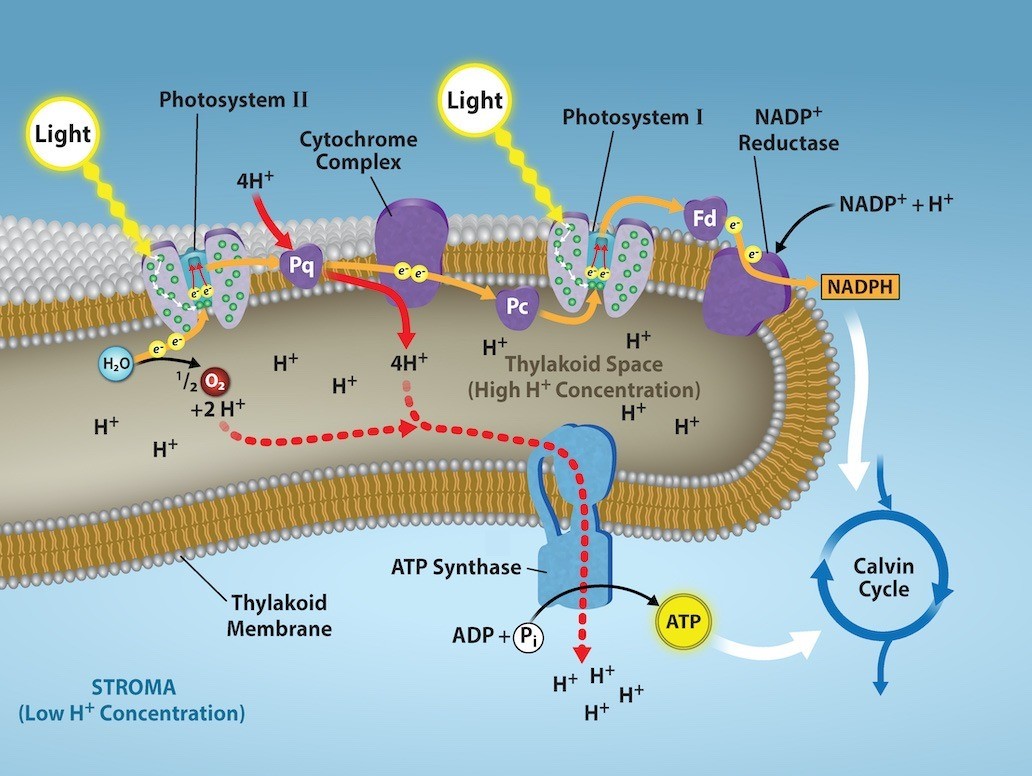
The Electron Transport Chain of Photosynthesis
After PSII extracts electrons from water, the subsequent steps involve getting those electrons onto the energy carrier NADPH for delivery to the Calvin cycle where the electron is deposited onto carbon for long-term storage in the form of a carbohydrate. PSII and PSI are two major components of the photosynthetic electron transport chain, which also includes the cytochrome complex. The cytochrome complex, an enzyme composed of two protein complexes, transfers the electrons from the carrier molecule plastoquinone (Pq) to the protein plastocyanin (Pc), thus enabling both the transfer of protons across the thylakoid membrane and the transfer of electrons from PSII to PSI.
The reaction center of PSII (called P680) delivers its high-energy electrons, one at the time, to the primary electron acceptor, and through the electron transport chain (Pq to cytochrome complex to plastocyanine) to PSI. P680’s missing electron is replaced by extracting a low-energy electron from water; thus, water is “split” during this stage of photosynthesis, and PSII is re-reduced each time it gives up an electron.
Splitting one H2O molecule releases two electrons, two hydrogen atoms, and one atom of oxygen. However, splitting two molecules is required to form one molecule of diatomic O2 gas. This oxygen byproduct is release into the atmosphere.
Each time an electron is moved from one molecule to another without an input of energy from light, some energy is lost (because an electron can only move from a higher energy state to a lower energy state without energy input). Therefore, as electrons move through the proteins that reside between PSII and PSI, they lose energy. The energy released from passing electrons from higher to lower energy states is harnessed to move hydrogen atoms from the stromal side of the membrane into the thylakoid lumen (Figure 23.12). Those hydrogen atoms, plus the ones produced by splitting water, accumulate in the thylakoid lumen and will be used synthesize ATP by ATP synthase in a process called chemiosmosis. Because the electrons have lost energy prior to their arrival at PSI, they must be re-energized by PSI, hence, another photon is absorbed by the PSI antenna. That energy is relayed to the PSI reaction center (called P700). P700 is oxidized and sends a high-energy electron to NADP+ to form NADPH. Thus, the energy captured from sunlight at PSII is used to create proton gradients to make ATP. Then, the energy captures from sunlight at PSI is used to reduce NADP+ into NADPH, which goes on to power the light-independent reactions of photosynthesis.
Chemiosmosis
Chemiosmosis is the process through which the Electron Transport Chain generates ATP. The energy harvested from sunlight by PSII is used to pump hydrogen ions inside the thylakoid lumen, creating a higher concentration of hydrogen ions inside the thylakoid than in the stroma (FIgure 23.12). The high concentration of charged ions means that there is both a concentration gradient as well as a charge gradient (the positive charges of the hydrogen ions repel each other). To release this energy, hydrogen ions will rush through any opening, similar to water jetting through a hole in a dam. In the thylakoid, that opening is a passage through a specialized protein channel called the ATP synthase. This strong electrochemical gradient means that hydrogen ions moving from high concentration (in the thylakoid lumen) to low concentration (in the stroma) through ATP synthase will release energy. The energy released by the hydrogen ion stream allows ATP synthase to attach a third phosphate group to ADP, which forms a molecule of ATP (Figure 23.12). The flow of hydrogen ions through ATP synthase is called chemiosmosis because the ions move from an area of high to an area of low concentration through a semipermeable structure of the thylakoid.
Reading Question #4
What is light energy directly used for in the first step of photosynthesis?
A. To phosphorylate ADP to generate ATP.
B. To extract electrons from water molecules.
C. To reduce NADPH.
D. To form covalent bonds between carbon atoms to create glucose.
Reading Question #5
What is the primary function of the light-dependent reactions in photosynthesis?
A. To convert solar energy into chemical energy in the form of NADPH and ATP
B. To convert carbon dioxide into glucose
C. To produce oxygen as a waste product
D. To break down carbohydrates into simple sugar
References and Acknowledgements
Pizza, R. (2020, November 24) Marie Clark Taylor: Exposing plants to different day lengths changes their flower development. Project Biodiversity. Retrieved May 27, 2022 from https://projectbiodiversify.org/2020/11/24/marie-clark-taylor/
Quinlisk, K. (2017, September 20) Fordham’s First Female Ph.D.. The Fordham Ram. Retrieved May 27, 2022 from https://thefordhamram.com/56782/culture/fordhams-first-female-ph-d/
Text adapted from Clark, M.A., Douglas, M., and Choi, J. (2018). Biology 2e. OpenStax. Retrieved from https://openstax.org/books/biology-2e/pages/1-introduction