24 Chapter 24: Photosynthesis: Light-independent reactions
Lisa Limeri
Learning Objectives
By the end of this section, students will be able to:
- Explain 1) the general role of ATP in the cell, 2) what it means to say that two chemical reactions are coupled, and 3) why a large change in free energy level occurs when an enzyme or substrate is phosphorylated. (Recall that phosphorylation adds 2 tightly packed negative charges.)
- Given a graph showing how free energy changes over the course of a chemical reaction, 1) label the sections representing the reactants, activation energy, transition state, and products, 2) describe and annotate to show how an enzyme would affects the energy curve, and 3) determine whether the reaction is exergonic or endergonic.
- Explain why enzymes increase reaction rates but do not make endergonic reactions exergonic.
Create a model that describes how plants “fix” carbon from the air to create sugars. Key terms your model should include: CO2, thylakoid, stroma, rubisco, NADP+/NADPH, ADP/ATP, Calvin Cycle, glucose, reduction, oxidation. - Predict the possible consequences for the production of ATP and NADPH if a component or process in the photosynthesis pathway is altered.
Introduction
In the last chapter, we learned about how the light-dependent reactions of photosynthesis convert light energy into chemical energy. However, the products of the light-dependent reactions, ATP and NADPH, are unstable forms of chemical energy and not suitable for long term storage or transfer. The light-independent reactions of photosynthesis use the energy stored in ATP and NADPH to synthesize carbohydrates, which are very stable and efficient at storing energy. Carbohydrates can be moved to different parts of the plant, stored as long as needed, and then broken down to provide useful energy to cells.
Before we can dive into the details of the light-independent reactions, we must first understand more about forms of energy and how energy levels change through chemical reactions.
Types of Energy
Energy is the ability to do work. Energy exists in different forms, e.g., electrical energy, light energy, and heat energy. In order to appreciate the way energy flows into and out of biological systems, it is important to understand more about the different energy types that exist in the physical world.
When an object is in motion, there is energy. For example, an airplane in flight produces considerable energy. This is because moving objects are capable of enacting a change, or doing work. Think of a wrecking ball. Even a slow-moving wrecking ball can do considerable damage to other objects. However, a wrecking ball that is not in motion is incapable of performing work. Energy with objects in motion is kinetic energy. A speeding bullet, a walking person, rapid molecule movement in the air (which produces heat), and electromagnetic radiation like light all have kinetic energy.
What if we lift that same motionless wrecking ball two stories above a car with a crane? If the suspended wrecking ball is unmoving, can we associate energy with it? Yes, the suspended wrecking ball has associated energy that is fundamentally different from the kinetic energy of objects in motion. This energy form results from the potential for the wrecking ball to do work. If we release the ball it would do work. Because this energy type refers to the potential to do work, we call it potential energy. Objects transfer their energy between kinetic and potential in the following way: As the wrecking ball hangs motionless, it has 0% kinetic and 100% potential energy. Once it releases, its kinetic energy begins to increase because it builds speed due to gravity. Simultaneously, as it nears the ground, it loses potential energy. Somewhere midfall it has 50% kinetic and 50% potential energy. Just before it hits the ground, the ball has nearly lost its potential energy and has near-maximal kinetic energy. Other examples of potential energy include water’s energy held behind a dam or a person about to skydive from an airplane.
We associate potential energy not only with the matter’s location, but also with the matter’s structure. A spring on the ground has potential energy if it is compressed; so does a tautly pulled rubber band. The very existence of living cells relies heavily on structural potential energy. On a chemical level, the bonds that hold the molecules’ atoms together have potential energy. Metabolic pathways that synthesize complex molecules from simple ones require energy (called anabolic pathways), and metabolic pathways release energy when complex molecules break down (called catabolic pathways). There is potential energy stored within the bonds of all the food molecules we eat, which we eventually harness for use. That energy originally comes from photosynthesis converting energy from sunlight into chemical energy in the form of the bonds in carbohydrates. This is because these bonds hold potential energy, and the molecules are in a lower energy state after that bond is broken. The potential energy within chemical bonds is chemical energy (Figure 24.1).
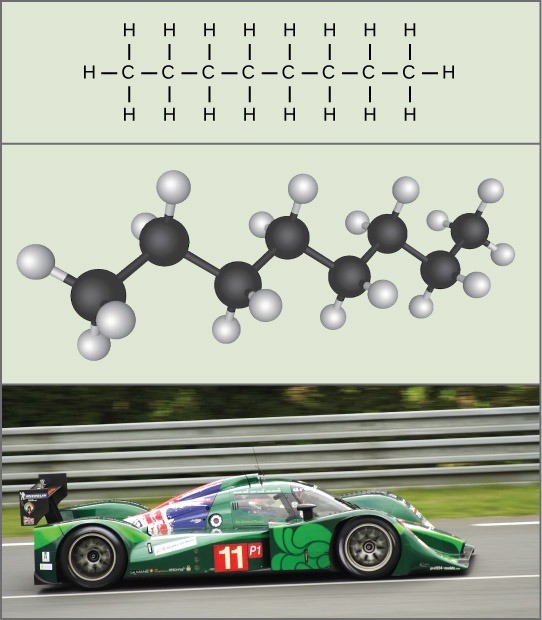
After learning that chemical reactions release energy when energy-storing bonds break, an important next question is how do we quantify and express the chemical reactions with the associated energy? How can we compare the energy that releases from one reaction to that of another reaction? We use a measurement of free energy to quantify these energy transfers: Gibbs free energy (abbreviated with the letter G), named after Josiah Willard Gibbs, the scientist who developed the measurement. Recall that according to the second law of thermodynamics, all energy transfers involve losing some energy in an unusable form such as heat, resulting in entropy. Gibbs free energy specifically refers to the energy that takes place with a chemical reaction that is available after we account for entropy. In other words, Gibbs free energy is usable energy, or energy that is available to do work. Every chemical reaction involves a change in free energy, called delta G (∆G). We can calculate the change in free energy for any system that undergoes such a change, such as a chemical reaction.
Endergonic Reactions and Exergonic Reactions
If energy is released during a chemical reaction, then the ∆G < 0. A negative ∆G also means that the reaction’s products have less free energy than the reactants, because they gave off some free energy during the reaction. Scientists call reactions that have a negative ∆G and consequently release free energy exergonic reactions or spontaneous reactions (Figure 24.2). Think: exergonic means energy is exiting the system. We also refer to these reactions as spontaneous reactions, because they can occur without adding energy into the system. Understanding which chemical reactions are spontaneous and release free energy is extremely useful for biologists, because these reactions can be harnessed to perform work inside the cell. We must draw an important distinction between the term spontaneous and the idea of a chemical reaction that occurs immediately. Contrary to the everyday use of the term, a spontaneous reaction is not one that suddenly or quickly occurs. Rusting iron is an example of a spontaneous reaction that occurs slowly over long periods of time.
Free energy diagrams illustrate the energy profiles for a given reaction (Figure 24.2). If a chemical reaction requires an energy input rather than releasing energy, then the ∆G for that reaction will be a positive value. In this case, the products have more free energy than the reactants. Thus, we can think of the reactions’ products as energy-storing molecules (Figure 24.2). We call these chemical reactions endergonic reactions, and they are non-spontaneous. An endergonic reaction will not take place on its own without adding free energy.
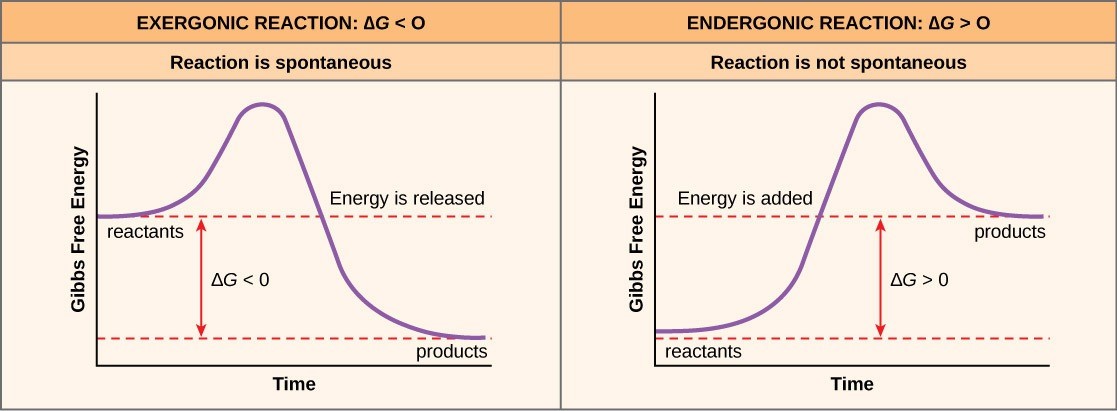
Let’s revisit the example of the synthesis and breakdown of the food molecule, glucose. Remember that building complex molecules, such as sugars, from simpler ones is an anabolic process and requires energy. Therefore, the chemical reactions involved in anabolic processes are endergonic reactions. Alternatively the catabolic process of breaking sugar down into simpler molecules releases energy in a series of exergonic reactions. Like the rust example above, the sugar breakdown involves spontaneous reactions, but these reactions do not occur instantaneously.
So how do cells get endergonic reactions to occur? There must be an input of energy into the system in order for an endergonic reaction to occur. This often occurs through reaction coupling, where an endergonic reaction is paired together with an exergonic reaction, so that energy released from the exergonic reaction is used to power the endergonic reaction. A common example is the hydrolysis of ATP, which is a highly exergonic reaction that releases a large amount of energy. This energy is often coupled to power an endergonic reaction.
Reading Question #1
Which type of reaction releases energy and is often referred to as “spontaneous”?
A. Endergonic reaction
B. Exergonic reaction
C. Anabolic reaction
D. Catabolic reaction
Activation Energy
There is another important concept that we must consider regarding endergonic and exergonic reactions. Even exergonic reactions require a small amount of energy input before they can proceed with their energy releasing steps. These reactions have a net release of energy, but still require some initial energy. Scientists call this small amount of energy input necessary for all chemical reactions to occur the activation energy abbreviated as “Ea” (Figure 24.3).
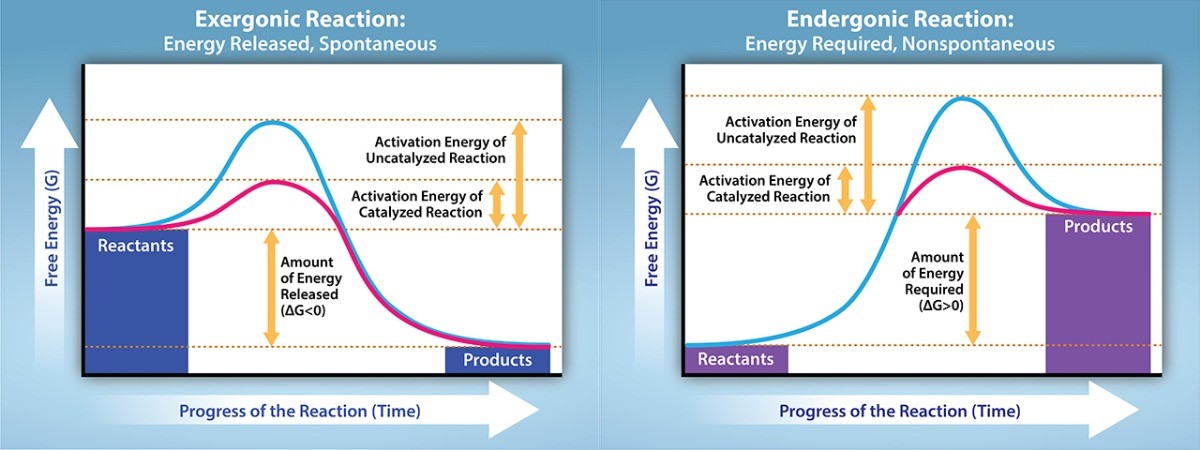
Why would an energy-releasing, negative ∆G reaction actually require some energy to proceed? The reason lies in the steps that take place during a chemical reaction. During chemical reactions, certain chemical bonds break and new ones form. For example, when a glucose molecule breaks down, bonds between the molecule’s carbon atoms break. Since these bonds ave high potential energy, they release energy when broken. However, to get them into a state that allows the bonds to break, the molecule must be somewhat contorted. A small energy input is required to achieve this contorted state. This contorted state is the transition state, and it is a high-energy, unstable state. For this reason, reactant molecules do not last long in their transition state, but very quickly proceed to the chemical reaction’s next steps. Whether the reaction is exergonic or endergonic determines whether the products in the diagram exist at a lower or higher energy state than the reactants. However, regardless of this measure, the transition state of the reaction exists at a higher energy state than the reactants, and thus, activation energy (Ea) is always positive.
The activation energy of a particular reaction determines the rate at which it will proceed. The higher the activation energy, the slower the chemical reaction. The example of iron rusting illustrates a spontaneous reaction that occurs slowly because of its high activation energy. Additionally, burning many fuels, which is strongly exergonic, will take place at a negligible rate unless sufficient heat from a spark overcomes their activation energy. However, once they begin to burn, the chemical reactions release enough heat to continue the burning process, supplying the activation energy for surrounding fuel molecules.
Enzymes Catalyze Reactions
The activation energy for most cellular reactions is too high for heat energy to overcome at efficient rates. In other words, in order for important cellular reactions to occur at appreciable rates (number of reactions per unit time), their activation energies must be lowered (Figure 24.3). This is called catalysis. Most often, a class of proteins called enzymes function to catalyze reactions by lowering the activation energy and make them happen much more readily than they would otherwise. Enzymes lower the activation energy by binding to the reactant molecules, and holding them in such a way as to stabilize and make it easier for reactants to reach the transition state and proceed through the reaction. Thus, enzymes speed up the rate of reactions by lowering the activation energy. It is important to note that enzymes do not affect the energy level of either the reactants or products. Therefore, enzymes do not affect whether a reaction is exergonic or endergonic.
Reading Question #2
What is activation energy?
A. The difference in energy between the reactants and the products.
B. The different in energy between the transition state and the products.
C. The different in energy between the reactants and the transition state.
D. The free energy of the reaction.
Enzyme Active Site and Substrate Specificity
The chemical reactants to which an enzyme binds are the enzyme’s substrates. There may be one or more substrates, depending on the particular chemical reaction. The location within the enzyme where the substrate binds is the enzyme’s active site. This is where the “action” happens. In protein enzymes, there is a unique combination of amino acid side chains within the active site. Different properties characterize each side chain. These can be large or small, acidic or basic, hydrophilic or hydrophobic, positively or negatively charged, or neutral. The unique combination of amino acid residues, their positions, sequences, structures, and properties, creates a very specific chemical environment within the active site. This specific environment is suited to bind, albeit briefly, to a specific chemical substrate (or substrates). Due to this jigsaw puzzle-like match between an enzyme and its substrates, enzymes are known for their specificity. The “best fit” results from the shape and the amino acid functional group’s attraction to the substrate. There is a specifically matched enzyme for each substrate and, thus, for each chemical reaction.
For many years, scientists thought that enzyme-substrate binding took place in a simple “lock-and-key” fashion. This model asserted that the enzyme and substrate fit together perfectly in one instantaneous step. However, current research supports a more refined view scientists call induced fit (Figure 24.4). This model expands upon the lock-and-key model by describing a more dynamic interaction between enzyme and substrate. As the enzyme and substrate come together, their interaction causes a mild shift in the enzyme’s structure that confirms an ideal binding arrangement between the enzyme and the substrate’s transition state. This ideal binding maximizes the enzyme’s ability to catalyze its reaction.
When an enzyme binds its substrate, it forms an enzyme-substrate complex. This complex lowers the reaction’s activation energy and promotes its rapid progression in one of many ways. On a basic level, enzymes promote chemical reactions that involve more than one substrate by bringing the substrates together in an optimal orientation. The appropriate region (atoms and bonds) of one molecule is juxtaposed to the other molecule’s appropriate region with which it must react. Another way in which enzymes promote substrate reaction is by creating an optimal environment within the active site for the reaction to occur. Certain chemical reactions might proceed best in a slightly acidic or non-polar environment. The chemical properties that emerge from the particular arrangement of amino acid residues within an active site create the perfect environment for an enzyme’s specific substrates to react. After an enzyme catalyzes a reaction, it releases its product(s).
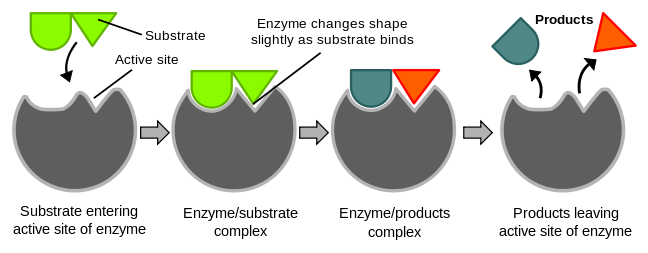
Energy stored in usable form as ATP in Living Systems
Cells store usable potential energy in the form of the molecule Adenosine Triphosphate (ATP). ATP is often called the “energy currency” of the cell, and, like currency, this versatile compound can be used to fill any energy need of the cell. It functions similarly to a rechargeable battery.
As the “tri” in the name implies, ATP has three phosphate groups attached to an adenosine. At the heart of ATP is a molecule of adenosine monophosphate (AMP), which is composed of an adenine nitrogenous base bonded to a ribose molecule and to a single phosphate group (Figure 24.5). AMP is one of the nucleotides in RNA. Phosphate groups are large and negatively charged, and thus repel each other. Three of them being bound together while strongly repelling each other is what gives ATP its high amount of potential energy (Figure 24.5). A large amount of this potential energy is released when ATP is broken down by the removal of its terminal phosphate group, called dephosphorylation. The energy is used to do work by the cell, usually when the released phosphate binds to another molecule, thereby increasing its potential energy. When the terminal phosphate group is removed, the molecule has only 2 phosphate groups and is called Adenosine Diphosphate (ADP). The molecule can be “recharged” by adding a third phosphate group – a phosphorylation. A reaction that adds a phosphate group to a molecule is called a phosphorylation reaction; a reaction that removes a phosphate from a molecule is called a dephosphorylation reaction.
Kinase is the name given to the group of enzymes that catalyze phosphorylation reactions. For example, in the mechanical work of muscle contraction, ATP supplies the energy to move the contractile muscle proteins. Recall the active transport work of the sodium-potassium pump in cell membranes. ATP alters the structure of the integral protein that functions as the pump, changing its affinity for sodium and potassium. In this way, the cell performs work, pumping ions against their electrochemical gradients.
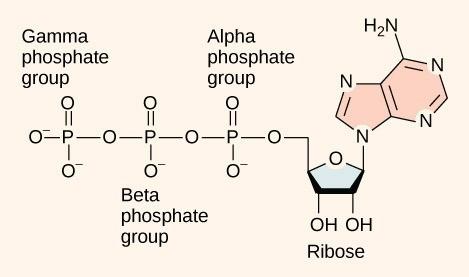
Hydrolysis is the process of using a water molecule to break complex macromolecules apart (hydro = water; lysis = to break apart). During hydrolysis, water is split, or lysed, and the resulting hydrogen atom (H+) and a hydroxyl group (OH–), or hydroxide, are added to the larger molecule. The hydrolysis of ATP produces ADP, an inorganic phosphate ion (Pi), and the release of free energy. To carry out life processes, ATP is continuously broken down into ADP, and like a rechargeable battery, ADP is continuously regenerated into ATP by the reattachment of a third phosphate group. Water, which was broken down into its hydrogen atom and hydroxyl group (hydroxide) during ATP hydrolysis, is regenerated when a third phosphate is added to the ADP molecule, reforming ATP.
The Light-Independent Reactions (a.k.a. the Calvin Cycle)
After the energy from the sun is converted into chemical energy and temporarily stored in ATP and NADPH molecules in the light-dependent reactions, the cell has the fuel needed to build carbohydrate molecules for long-term energy storage. The products of the light-dependent reactions, ATP and NADPH, have lifespans in the range of millionths of seconds, whereas the products of the light-independent reactions (carbohydrates and other forms of reduced carbon) can survive almost indefinitely. The carbohydrate molecules made will have a backbone of carbon atoms. But where does the carbon come from? It comes from carbon dioxide in the atmosphere.
In plants, carbon dioxide (CO2) enters the leaves through stomata, where it diffuses over short distances to the mesophyll cells (Fig 24.6). Once in the mesophyll cells, CO2 diffuses into the stroma of the chloroplast—the site of light-independent reactions of photosynthesis, which are collectively called the Calvin cycle (named for the researcher who discovered them) and because these reactions function as a cycle. .

The light-independent reactions of the Calvin cycle can be organized into three basic stages: fixation, reduction, and regeneration (Figure 24.7)

Calvin Cycle Phase 1: Carbon Fixation
In the stroma, in addition to CO2, two other components are present to initiate the light-independent reactions: an enzyme called RuBisCO (which is an acronym for its full name, ribulose-1,5-bisphosphate carboxylase/oxygenase), and three molecules of ribulose bisphosphate (RuBP) (Figure 24.8). RuBP has five atoms of carbon, flanked by two phosphates. RuBisCO catalyzes a reaction between CO2 and RuBP to form two molecules of 3-phosphoglycerate (3-PGA). Each 3-PGA has three carbons and one phosphate. Notice how the number of carbon atoms remains the same in this step (1 C atom from CO2 + 5 C atoms from RuBP = 6 C atoms in 2 molecules of 3-PGA). This process is called carbon fixation, because CO2 is “fixed” from a gaseous, inorganic form into organic molecules. The benefit of fixing CO2 is the carbon moving from its most oxidized (thus, lowest energy) state as CO2 to a much more reduced form of carbon, 3-PGA, and thus a higher energy state.
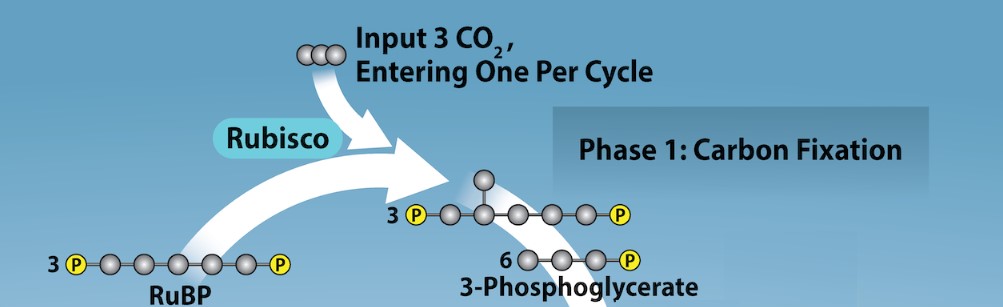
Reading Question #3
What does carbon fixation mean?
A. Carbon is converted from inorganic gaseous form to an organic molecule.
B. Carbon’s energy level is decreased.
C. Carbon is oxidized.
D. Carbon is absorbed from the soil and incorporated into plant tissue.
Calvin Cycle Phase 2: Reduction
ATP and NADPH, the products of the light-dependent reactions, are used to energize and reduce the six molecules of 3-PGA into six molecules of a chemical called glyceraldehyde 3-phosphate (G3P). In the first step of G3P production, ATP is hydrolyzed and its terminal phosphate is added to 3-PGA to form 1,3-bisposphoglycerate (Figure 24.9; first step). The addition of the phosphate is another in the goal of raising the free energy of this carbon intermediate. Next, 1,3-bisphosphoglycerate is reduced by the oxidation of NADPH to form G3P and NADP+(Figure 24.8; second step). This a highly endergonic reaction as it involves the addition of electrons to 1,3-bisphosphoglycerate to form G3P.
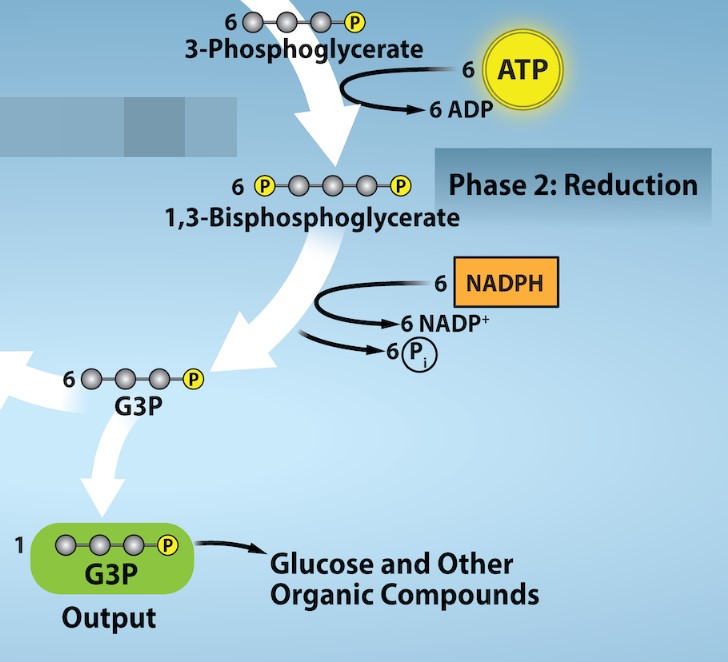
As we account for the inputs and outputs, we have to take into account that six CO2 molecules are fixed to form one six-carbon glucose. Therefore, we will require the hydrolysis of six molecules of ATP and the oxidation of six molecules of NADPH along the way in Phase 2: Reduction. The benefit of this phase is two-fold. The G3P products are used to form glucose and other organic molecules. In addition, the ADP and NADP+ regenerated along the way resupply the light-dependent reactions, allowing that process to continue. This highlights how both light-dependent and light-independent reactions are intertwined. The light-dependent reactions generate the high energy intermediates ATP and NADPH for carbon fixation and the light-independent reactions recycle the ADP and NADP+ to send back to the light-dependent reactions.
Reading Question #4
The reactions in the reduction phase of the Calvin Cycle require the input of energy and electrons. What is the direct source of energy and electrons for these reactions?
A. ATP from respiration in the mitochondria.
B. Light energy absorbed by chlorophyll.
C. The products of the light-dependent reactions, ATP and NADPH.
D. Rubisco.
Calvin Cycle Phase 3: Regeneration
The Calvin Cycle is called a cycle because the final stage regenerates the molecules that are needed as reactants in the initial, fixation stage (Fig 24.8). In order for carbon to be fixed, the cell needs RuBP molecules. If RuBP is always used but never replenished, the cell would run out and be unable to continue synthesizing carbohydrates. So, the cell uses some of the G3P molecules produced from the reduction stage to regenerate RuBP.
After the reduction stage, only one of the G3P molecules leaves the Calvin cycle and is sent to the cytoplasm to contribute to the formation of other compounds needed by the plant (such as glucose). Because the G3P exported from the chloroplast has three carbon atoms, it takes three “turns” of the Calvin cycle to fix enough net carbon to export one G3P. But each turn makes two G3Ps, thus three turns make six G3Ps. One is exported while the remaining five G3P molecules remain in the cycle and are used to regenerate RuBP, which enables the system to prepare for more CO2 to be fixed (Figure 24.10). Regenerating the reactants to fix more CO2 is energetically costly; the cell must use three more molecules of ATP in these regeneration reactions.
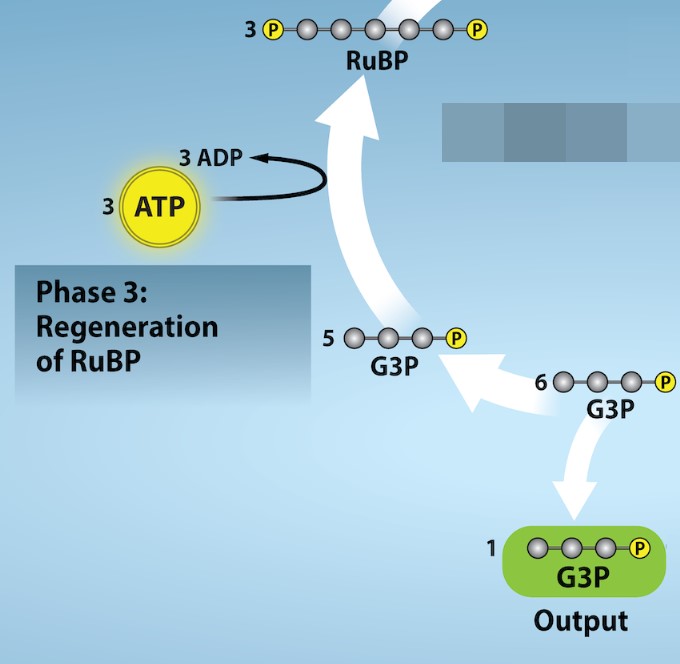
Evolution Connection: Photosynthesis
During the evolution of photosynthesis, a major shift occurred from the bacterial type of photosynthesis that involves only one photosystem and is typically anoxygenic (does not generate oxygen) into modern oxygenic (does generate oxygen) photosynthesis, employing two photosystems.
This modern oxygenic photosynthesis is used by many organisms—from giant tropical leaves in the rainforest to tiny cyanobacterial cells—and the process and components of this photosynthesis remain largely the same. Photosystems absorb light and use electron transport chains to convert energy into the chemical energy of ATP and NADPH. The subsequent light independent reactions then assemble carbohydrate molecules with this energy.
In the harsh dry heat of the desert, plants must conserve and use every drop of water to survive. They have evolved processes to conserve water in these harsh conditions. Stomata must open to allow for the uptake of CO2, but when they open, water escapes from the leaf during active photosynthesis. So, these desert plants evolved an enhanced form of photosynthesis called crassulacean acid metabolism (CAM), where they can open their stomata at night, conserving water due to cooler temperatures. CAM plants such as cacti (Figure 24.11) and succulents can prepare materials for photosynthesis overnight using a temporary carbon fixation/storage process (University of Liverpool, 2017). During the day, the plants use the captured CO2 for photosynthesis and keep their stomata closed.
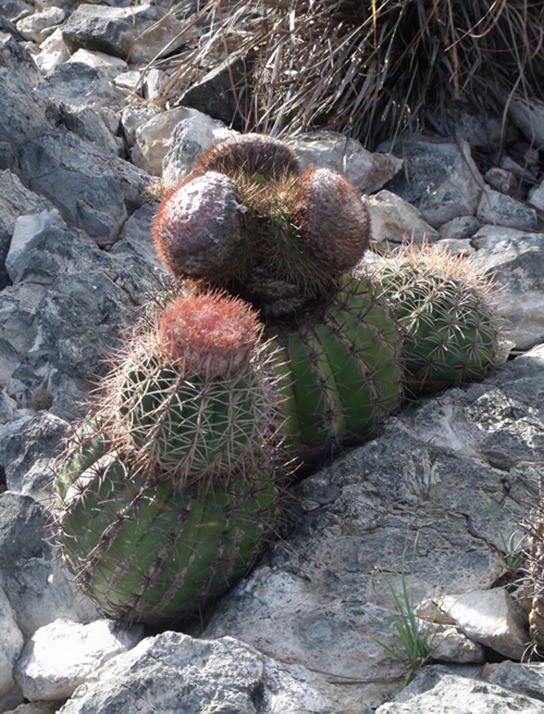
Genetic engineering efforts are trying to create crops that are more drought-resistant, making CAM a subject of interest. Recent research has determined that the enzyme PPCK is a necessary component of CAM photosynthesis. In order for CAM photosynthesis to work properly, plant cells must switch on PPCK every night, as driven by their internal circadian clock (University of Liverpool, 2017).
Reading Question #5
What is the primary function of the Calvin cycle in photosynthesis?
A. To produce ATP and NADPH.
B. To convert light energy into chemical energy.
C. To fix carbon dioxide (CO2) into reduced, high-energy, stable, organic molecules.
D. To release oxygen (O2) into the atmosphere.
The Energy Flow
Whether the organism is a bacterium, plant, or animal, all living things access energy by breaking down carbohydrate and other carbon-rich organic molecules. But if plants make carbohydrate molecules, why would they need to break them down, especially when it has been shown that the gas organisms release as a “waste product” (CO2) acts as a substrate for the formation of more food in photosynthesis? Remember, living things need energy to perform life functions. In addition, an organism can either make its own food or eat another organism—either way, the food still needs to be broken down. Finally, in the process of breaking down food, called cellular respiration, heterotrophs release needed energy and produce “waste” in the form of CO2 gas.
However, in nature, there is no such thing as “waste.” Every single atom of matter and energy is conserved, recycled over and over infinitely. Substances change form or move from one type of molecule to another, but their constituent atoms never disappear. In reality, CO2 is no more a form of waste than oxygen is wasteful to photosynthesis. Both are byproducts of reactions that move on to other reactions. Photosynthesis absorbs light energy to build carbohydrates in chloroplasts, and aerobic cellular respiration releases energy by using oxygen to metabolize carbohydrates in the cytoplasm and mitochondria. Both processes use electron transport chains to capture the energy necessary to drive other reactions. These two powerhouse processes, photosynthesis and cellular respiration, function in biological, cyclical harmony to allow organisms to access life-sustaining energy that originates millions of miles away in a burning star humans call the sun.
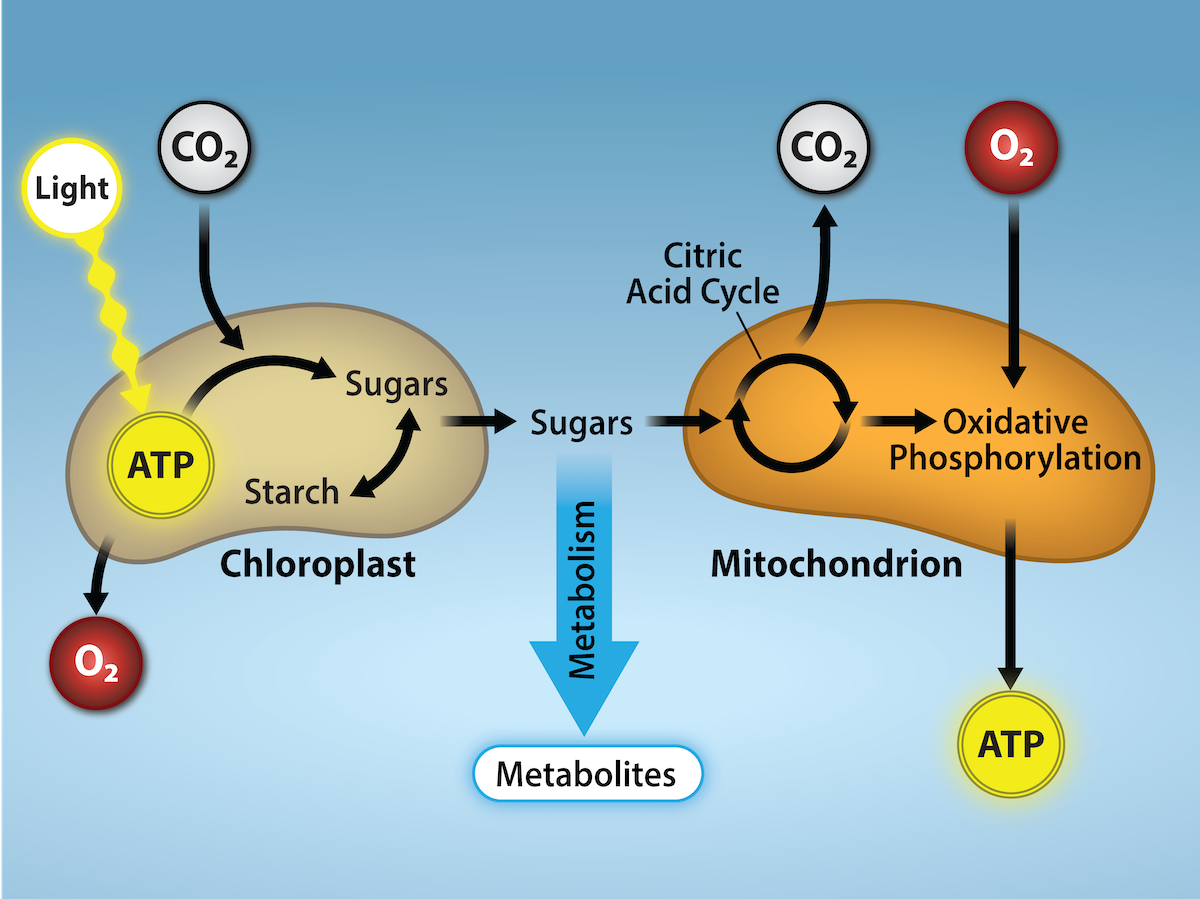
References and Acknowledgements
Adapted from Clark, M.A., Douglas, M., and Choi, J. (2018). Biology 2e. OpenStax. Retrieved from https://openstax.org/books/biology-2e/pages/1-introduction