29 Chapter 29: Energy Sources Carbohydrates and Lipids
Lisa Limeri
Learning Objectives
By the end of this section, students will be able to:
- Compare the monomer subunit, bond responsible for polymerization, and important biological function(s) observed in proteins, fats, nucleic acids, and carbohydrates.
- Compare the structures, functions, and energy potential of unsaturated fats, saturated fats, triglycerides, and carbohydrates.
Introduction
Food provides the body with the nutrients it needs to survive. Many of these critical nutrients are biological macromolecules, or large molecules, necessary for life. Macromolecules are a subset of organic molecules (carbon-containing molecules) that are especially important for life. Different smaller organic molecule (monomer) combinations build these macromolecules (polymers). What specific biological macromolecules do living things require? How do these molecules form? What functions do they serve? We explore these questions in this chapter.
Biological macromolecules are large molecules, necessary for life, that are built from smaller organic molecules. There are four major biological macromolecule classes (carbohydrates, lipids, proteins, and nucleic acids). Each is an important cell component and performs a wide array of functions. Combined, these molecules make up the majority of a cell’s dry mass (recall that water makes up the majority of its complete mass). Biological macromolecules are organic, meaning they contain carbon. In addition, they may contain hydrogen, oxygen, nitrogen, and additional minor elements.
We have already discussed two important classes of biological macromolecules in this course: nucleic acids and proteins. Today, we will learn about two more important classes: carbohydrates and lipids.
Synthesis of Biological Macromolecules
Dehydration Synthesis
Most macromolecules are made from single subunits, or building blocks, called monomers. The monomers combine with each other using covalent bonds to form larger molecules known as polymers. In doing so, monomers release water molecules as byproducts. This type of reaction is dehydration synthesis, which means “to put together while losing water.”
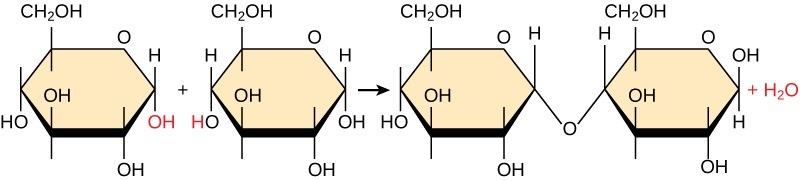
In a dehydration synthesis reaction (Figure 29.1), the hydrogen (H+) of one monomer combines with the hydroxyl group (OH–) of another monomer, releasing a water molecule (H20). At the same time, the monomers share electrons and form covalent bonds. As additional monomers join, this chain of repeating monomers forms a polymer. Different monomer types can combine in many configurations, giving rise to a diverse group of macromolecules. Even one kind of monomer can combine in a variety of ways to form several different polymers. For example, glucose monomers are the constituents of starch, glycogen, and cellulose.
Reading Question #1
Which of the following describes a dehydration synthesis?
A. Combines monomers to create a polymer and generates water as a product.
B. Combines polymers to create a monomer and generates water as a product.
C. Combines monomers to synthesize a polymer and uses water as a reactant.
D. Breaks apart a polymer into monomers and uses water as a reactant.
Hydrolysis
Polymers break down into monomers during hydrolysis: a chemical reaction in which inserting a water molecule breaks a covalent bond (Figure 29.2). During these reactions, the polymer breaks into two components: one part gains a hydrogen atom (H+) and the other gains a hydroxyl molecule (OH–) from a split water molecule.
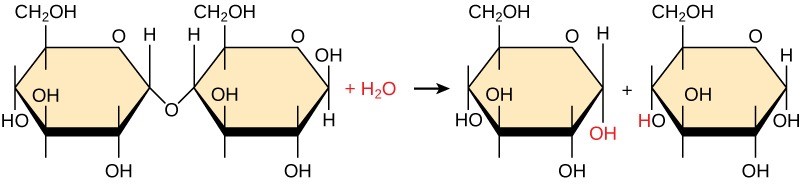
Dehydration and hydrolysis reactions are catalyzed by specific enzymes; dehydration reactions involve the formation of new bonds, requiring energy, while hydrolysis reactions break bonds and release energy. These reactions are similar for most macromolecules, but each monomer and polymer reaction is specific for its class. For example, catalytic enzymes in the digestive system hydrolyze or break down the food we ingest into smaller molecules. This allows cells in our body to easily absorb nutrients in the intestine. A specific enzyme breaks down each macromolecule. For instance, amylase, sucrase, lactase, or maltase break down carbohydrates. Enzymes called proteases, such as pepsin and peptidase, and hydrochloric acid break down proteins. Lipases break down lipids. These broken down macromolecules provide energy for cellular activities.
Reading Question #2
Which of the following describes a hydrolysis reaction?
A. Combines monomers to create a polymer and generates water as a product.
B. Breaks apart a polymer into monomers and generates water as a product.
C. Combines monomers to synthesize a polymer and uses water as a reactant.
D. Breaks apart a polymer into monomers and uses water as a reactant.
Structure and Function of Macromolecules
Carbon
The fundamental component for all of these macromolecules is carbon. The carbon atom has unique properties that allow it to form covalent bonds to 4 atoms, making this versatile element ideal to serve as the basic structural component, or “backbone,” of the macromolecules. Due to their chemical properties, carbon atoms almost always have four covalent bonds in molecules.
Hydrocarbons
Hydrocarbons are organic molecules consisting entirely of carbon and hydrogen, such as methane (CH4). We often use hydrocarbons in our daily lives as fuels—like the propane in a gas grill or the butane in a lighter. The many covalent bonds between the atoms in hydrocarbons store a great amount of energy, which releases when these molecules burn (oxidize). Methane, an excellent fuel, is the simplest hydrocarbon molecule, with a central carbon atom bonded to four hydrogen atoms.
As the backbone of the large molecules of living things, hydrocarbons may exist as linear carbon chains, carbon rings, or combinations of both. Carbon-to-carbon bonds may be single, double, or triple covalent bonds, and each type of bond affects the molecule’s geometry in a specific way. This three-dimensional shape or conformation of the large molecules of life (macromolecules) is critical to how they function.
Successive bonds between carbon atoms form hydrocarbon chains. These may be branched or unbranched, and this is influenced by whether there are single, double, and triple covalent bonds between carbons in the chain. Single bonds result in straight chains whereas double and triple bonds introduce bends or kinks in the chain. You may remember from the diffusion module that the straightness or crookedness of fatty acid chains impacts the plasma membrane’s structure and function.
Isomers
The three-dimensional placement of atoms and chemical bonds within organic molecules is central to understanding their biochemistry. We call molecules that share the same chemical formula but differ in the placement (structure) of their atoms and/or chemical bonds isomers. Structural isomers (like butane and isobutane in Figure 29.3a) differ in the placement of their covalent bonds: both molecules have four carbons and ten hydrogens (C4H10), but the different atom arrangement within the molecules leads to differences in their chemical properties. For example, butane is suited for use as a fuel for cigarette lighters and torches; whereas, isobutane is suited for use as a refrigerant and a propellant in spray cans.
Geometric isomers, alternatively have similar placements of their covalent bonds but differ in how these bonds are made to the surrounding atoms, especially in carbon-to-carbon double bonds. In the simple molecule butene (C4H8), the two methyl groups (CH3) can be on either side of the double covalent bond central to the molecule, as Figure 29.3b illustrates. When the carbons are bound on the same side of the double bond, this is the cis configuration. If they are on opposite sides of the double bond, it is a trans configuration. In the trans configuration, the carbons form a more or less linear structure; whereas, the carbons in the cis configuration make a bend (change in direction) of the carbon backbone.
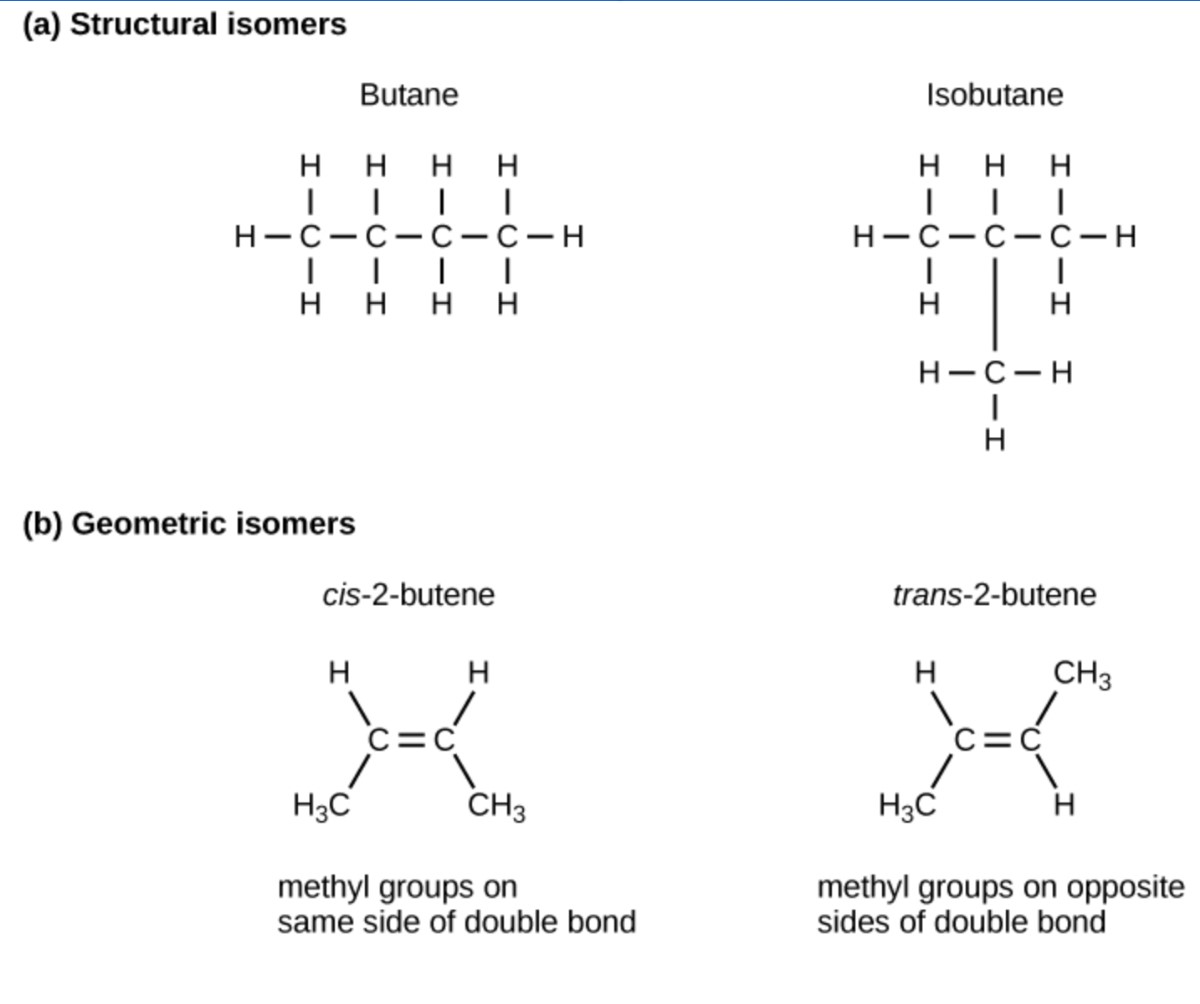
Functional Groups
Functional groups are groups of atoms that occur within molecules and confer specific chemical properties to those molecules. The functional groups in a macromolecule are usually attached to the carbon backbone at one or several different places along its chain and/or ring structure. Each of the four types of macromolecules—proteins, lipids, carbohydrates, and nucleic acids—has its own characteristic set of functional groups that contributes greatly to its differing chemical properties and its function in living organisms.
A functional group can participate in specific chemical reactions. Table 29.1 shows some of the important functional groups in biological molecules. They include: hydroxyl, methyl, carbonyl, carboxyl, amino, phosphate, and sulfhydryl. These groups play an important role in forming molecules like DNA, proteins, carbohydrates, and lipids. We usually classify functional groups as hydrophobic or hydrophilic depending on their charge or polarity characteristics. An example of a hydrophobic group is the nonpolar methyl molecule. Among the hydrophilic functional groups is the carboxyl group in amino acids, some amino acid side chains, and the fatty acids that form triglycerides and phospholipids. This carboxyl group ionizes to release hydrogen ions (H+) from the COOH group resulting in the negatively charged COO– group. This contributes to the hydrophilic nature of whatever molecule on which it is found. Other functional groups, such as the carbonyl group, have a partially negatively charged oxygen atom that may form hydrogen bonds with water molecules, again making the molecule more hydrophilic.
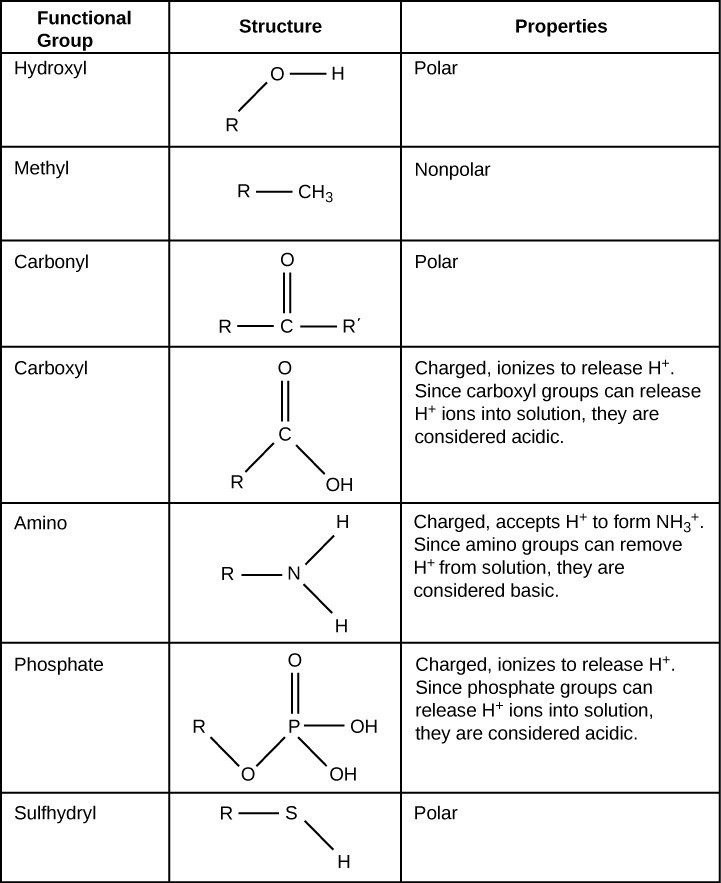
Hydrogen bonds between functional groups (within the same molecule or between different molecules) are important to the function of many macromolecules and help them to fold properly into and maintain the appropriate shape for functioning. Hydrogen bonds are also involved in various recognition processes, such as DNA complementary base pairing and the binding of an enzyme to its substrate, as Figure 29.4 illustrates.
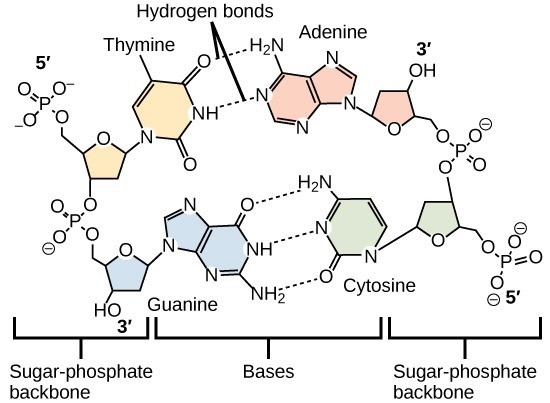
Carbohydrates
Most people are familiar with carbohydrates as they are an essential part of our diet. Grains, fruits, and vegetables are all natural carbohydrate sources that provide energy to the body, particularly through glucose, a simple sugar that is a component of starch and an ingredient in many staple foods. Carbohydrates also have other important functions in humans, animals, and plants.
Molecular Structures
The ratio of carbon to hydrogen to oxygen is 1:2:1 in carbohydrate molecules. This formula also explains the origin of the term “carbohydrate”: the components are carbon (“carbo”) and the components of water (hence, “hydrate”). Scientists classify carbohydrates into three subtypes: monosaccharides, disaccharides, and polysaccharides.
Monosaccharides (mono- = “one”; sacchar- = “sweet”) are simple sugars, the most common of which is glucose. Glucose is an important source of energy that is metabolized through cellular respiration. Galactose (part of lactose, or milk sugar) and fructose (found in sucrose, in fruit) are other common monosaccharides. Although glucose, galactose, and fructose all have the same chemical formula (C6H12O6), they differ structurally and chemically (and are thus isomers) because of the different arrangement of functional groups (Figure 29.5).
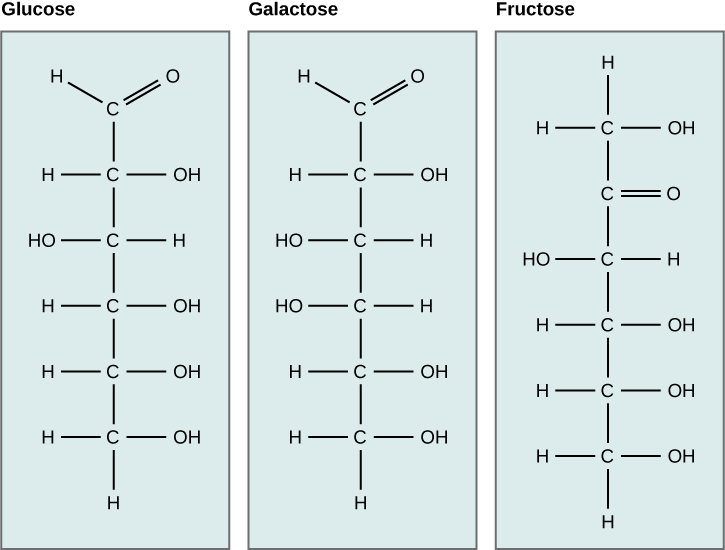
Disaccharides (di- = “two”) form when two monosaccharides are connected via a dehydration reaction. A bond linking two monosaccharides together to create a disaccharide is a glycosidic linkage or glycosidic bond (Figure 29.6). Common disaccharides include lactose, maltose, and sucrose. Lactose is a disaccharide consisting of the monomers glucose and galactose. It is naturally-occurring in milk. Maltose, or malt sugar, is a disaccharide formed by a dehydration reaction between two glucose molecules. The most common disaccharide is sucrose, or table sugar, which is comprised of glucose and fructose monomers.
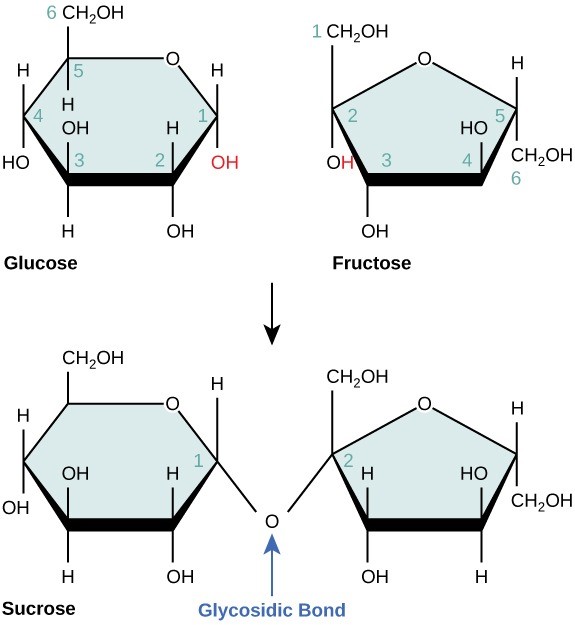
A long chain of monosaccharides linked by glycosidic bonds is a polysaccharide (poly- = “many”). The chain may be branched or unbranched, and it may contain different types of monosaccharides. Starch, glycogen, cellulose, and chitin are primary examples of polysaccharides.
Plants store sugars in the form of starch. In plants, an amylose and amylopectin mixture (both glucose polymers) comprise these sugars. Plants are able to synthesize glucose through photosynthesis, and they store the excess glucose as starch in different plant parts, including roots and seeds. The starch in the seeds provides food for the embryo as it germinates and can also act as a food source for humans and animals. Enzymes break down the starch that humans consume. For example, an amylase present in saliva catalyzes, or breaks down this starch into smaller molecules, such as maltose and glucose. The cells can then absorb the glucose. Unbranched glucose monomer chains form the starch; whereas, amylopectin is a branched polysaccharide (Figure 29.7).
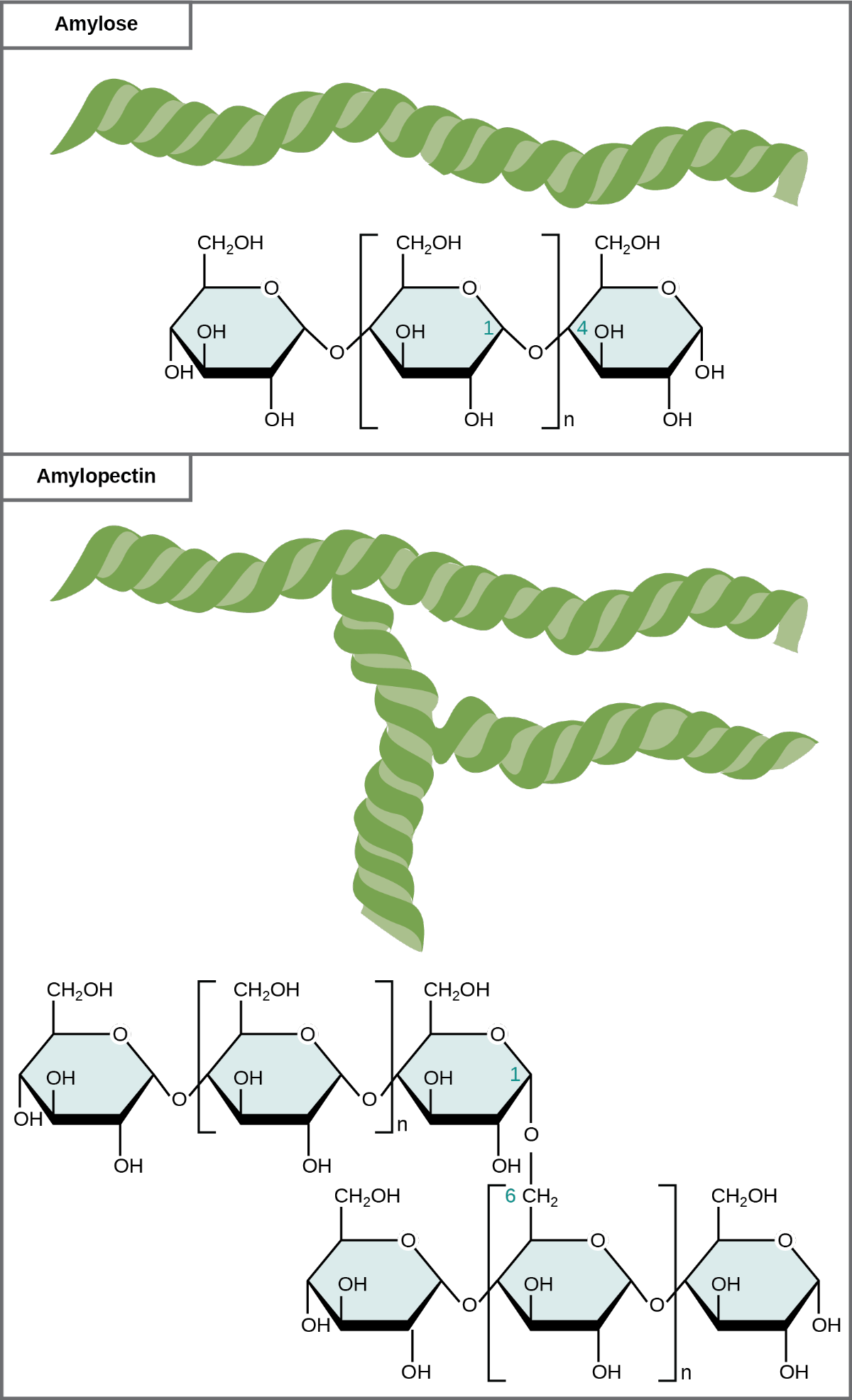
Glycogen is the storage form of glucose in humans and other vertebrates and is comprised of monomers of glucose. Glycogen is the animal equivalent of starch and is a highly branched molecule usually stored in liver and muscle cells. Whenever blood glucose levels decrease, glycogen is broken down to release glucose.
Cellulose mostly comprises plants’ cell walls and is the most abundant natural polymer. Cellulose provides the cell structural support. Wood and paper are mostly composed of cellulose (Figure 29.8).
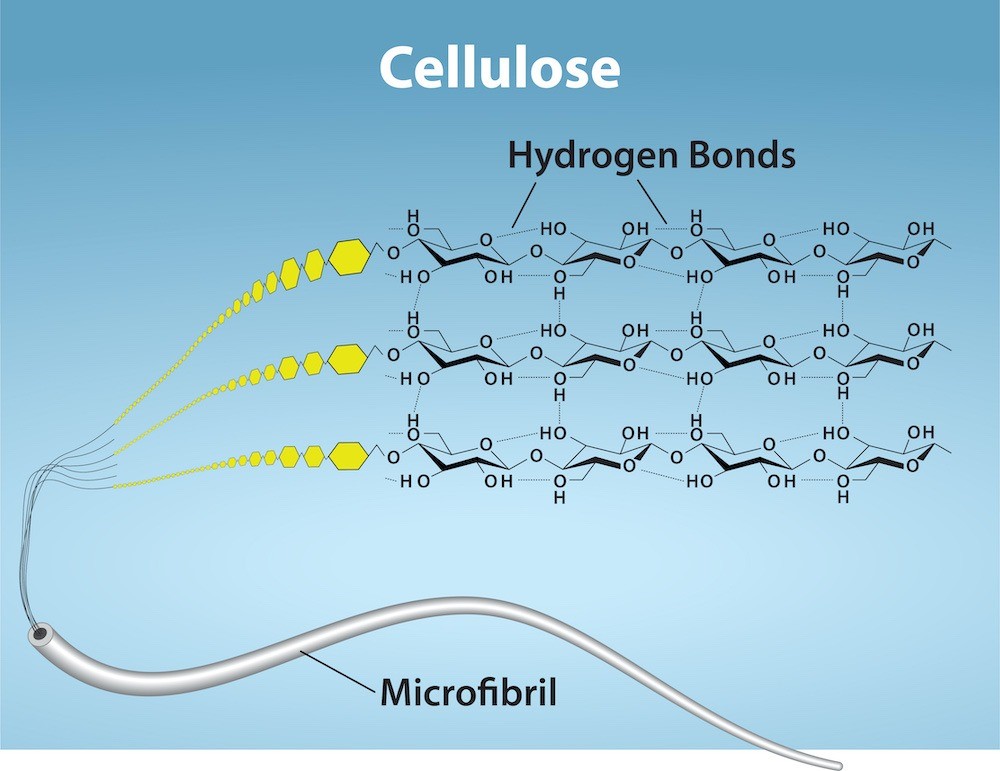
As Figure 29.8 above shows, every other glucose monomer in cellulose is flipped over, and the monomers are packed tightly as extended long chains. This gives cellulose its rigidity and high tensile strength—which is so important to plant cells. While human digestive enzymes cannot break down the glycosidic bonds that connect glucoses in cellulose, herbivores (ex. cows, koalas, and buffalos) have enzymes that can. This is due to specialized microbiota in their stomach, to digest plant material that is rich in cellulose and use it as a food source. In some of these animals, certain species of bacteria and protists reside in the rumen (part of the herbivore’s digestive system) and secrete the enzyme cellulase. The appendix of grazing animals also contains bacteria that digest cellulose, giving it an important role in ruminants’ digestive systems. Cellulases can break down cellulose into glucose monomers that animals use as an energy source. Termites are also able to break down cellulose because of the presence of other organisms in their bodies that secrete cellulases.
Arthropods (insects, crustaceans, and others) have an outer skeleton, the exoskeleton, which protects their internal body parts. This exoskeleton is made of chitin, which is a nitrogen-containing polysaccharide. It is made of repeating N-acetyl-β-dglucosamine units, which are a modified sugar. Chitin is also a major component of fungal cell walls. Fungi are neither animals nor plants and form a kingdom of their own in the domain Eukarya.
Reading Question #3
Which of the following describes a disaccharide?
A. Many polysaccharides linked together by an ester bond.
B. Two polysaccharides linked together by a glycosidic bond.
C. Two monosaccharides linked together by a glycosidic bond.
D. The monomer components of a polysaccharide.
Lipids
Lipids include a diverse group of compounds that are largely nonpolar in nature. This is because they are hydrocarbons that include mostly nonpolar carbon–carbon or carbon–hydrogen bonds. Non-polar molecules are hydrophobic (“water fearing”), or insoluble in water. Lipids perform many different functions in a cell. Cells store energy for long-term use in the form of fats. Lipids also provide insulation from the environment for plants and animals. For example, they help keep aquatic birds and mammals dry when forming a protective layer over fur or feathers because of their water-repellant hydrophobic nature. Lipids are also the building blocks of many hormones and are an important constituent of all cellular membranes. Lipids include fats, oils, waxes, phospholipids, and steroids.
Here we will focus on fats and oils, which primarily function in energy storage. Mammals store fats in specialized cells called adipocytes, where fat globules occupy most of the cell’s volume. Plants store fat or oil in many seeds and use them as a source of energy during seedling development.
Triglycerides (Fats)
A triglyceride (also called fat) molecule consists of two main components—glycerol and fatty acids. Glycerol is an organic alcohol with three carbons, five hydrogens, and three hydroxyl (OH) groups. Fatty acids have a long chain of hydrocarbons to which a carboxyl group is attached, hence the name “fatty acid.” In a fat molecule, the fatty acids attach to each of the glycerol molecule’s three carbons with an ester bond through an oxygen atom (Figure 29.9).
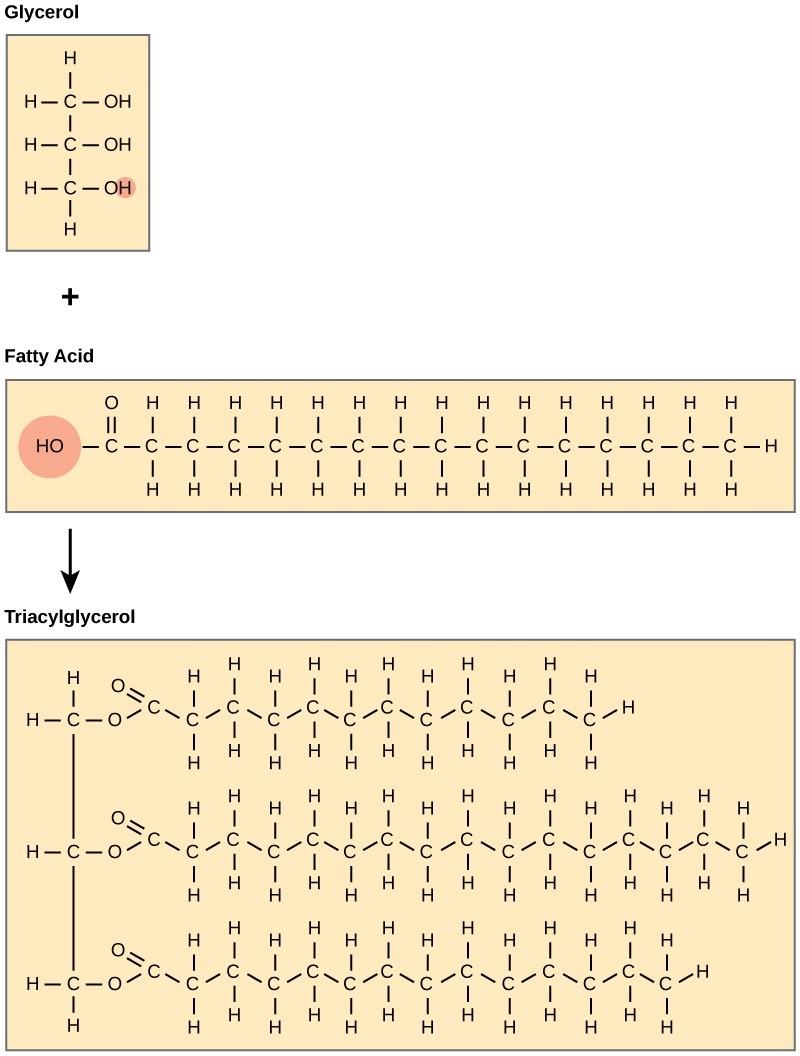
During this ester bond formation, three water molecules are released. The three fatty acids in the triacylglycerol may be similar or dissimilar. We also call fats triacylglycerols or triglycerides because of their chemical structure.
Fatty Acids
Fatty acids are a hydrocarbon chain attached to a carboxylic acid functional group. The hydrocarbon chain may be saturated or unsaturated. If the fatty acid chain consists of only single bonds between neighboring carbons, the fatty acid is saturated. Saturated fatty acids are saturated with hydrogen. In other words, the number of hydrogen atoms attached to the carbon skeleton is maximized. Stearic acid is an example of a saturated fatty acid (Figure 29.10).
In contrast, when the hydrocarbon chain contains one or more double bond, the fatty acid is unsaturated. Oleic acid is an example of an unsaturated fatty acid (Figure 29.10). Most unsaturated fats are liquid at room temperature. We call these oils. If there is only one double bond in the molecule, then it is a monounsaturated fat (e.g., olive oil), and if there is more than one double bond, then it is a polyunsaturated fat (e.g., canola oil).
Cis and trans indicate the configuration of the molecule around a double bond in an unsaturated fatty acid. If hydrogens are present in the same plane, it is a cis fat. The resulting bend in the chain’s carbon backbone means that triglyceride molecules cannot pack tightly, so they remain liquid (oil) at room temperature. If the hydrogen atoms are on two different planes, it is a trans fat. The cis double bond causes a bend or a “kink” that prevents the fatty acids from packing tightly, keeping them liquid at room temperature (Figure 29.10).
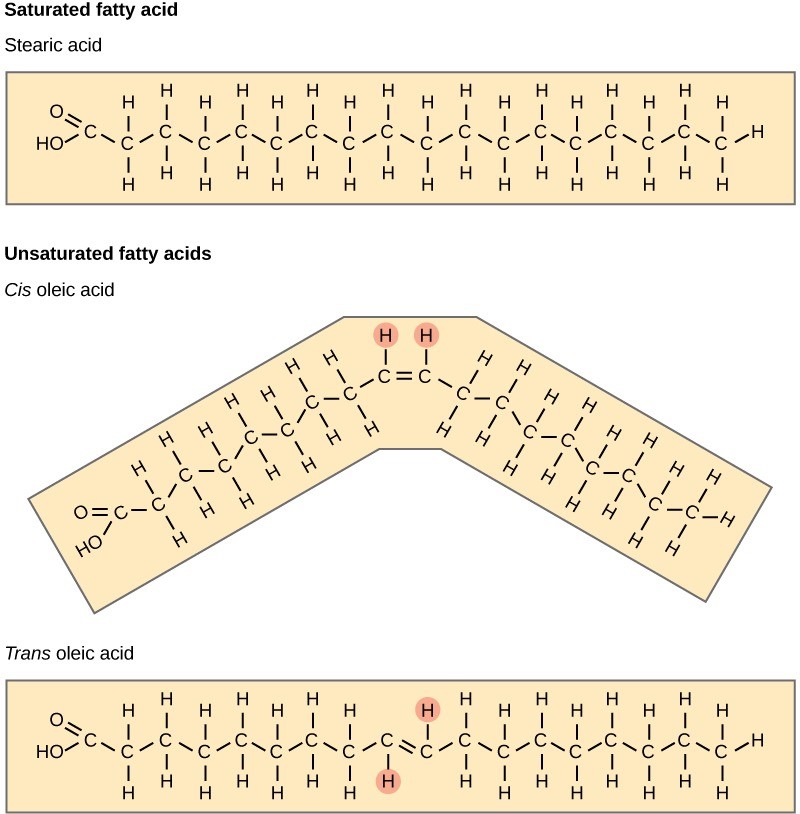
Everyday Connection: Fats and Nutrition
The food industry artificially hydrogenates oils to make them semi-solid and of a consistency desirable for many processed food products. Simply speaking, hydrogen gas is bubbled through oils to solidify them. During this hydrogenation process, double bonds of the cis– conformation in the hydrocarbon chain may convert to double bonds in the trans– conformation.
Margarine, some types of peanut butter, and shortening are examples of artificially hydrogenated trans fats. Studies have shown that an increase in trans fats in the human diet may lead to higher levels of low-density lipoproteins (LDL), or “bad” cholesterol, which in turn may lead to plaque deposition in the arteries, resulting in heart disease (Brouwer, Wanders, & Katan, 2010). As of 2021, 40 countries have banned the use of trans fats; these are all high-income countries, so people in low-income countries remain unprotected by best practice policies (WHO, 2021).
It is important to remember that we need fats in our diets. Essential fatty acids are those that the human body requires but does not synthesize. Consequently, they have to be supplemented through ingestion via the diet. Omega-3 fatty acids fall into this category and are one of only two known for humans (the other is omega-6 fatty acid). These are polyunsaturated fatty acids and are omega-3 because a double bond connects the third carbon from the hydrocarbon chain’s end to its neighboring carbon.
Salmon, trout, and tuna are good sources of omega-3 fatty acids. Research indicates that omega-3 fatty acids reduce the risk of sudden death from heart attacks, lower triglycerides in the blood, decrease blood pressure, and prevent thrombosis by inhibiting blood clotting. They also reduce inflammation, and may help lower the risk of some cancers in animals.
Reading Question #4
Which macromolecule is composed of a glycerol attached to three fatty acid chains that have straight hydrocarbon tails with all single bonds?
A. Saturated triglyceride
B. Carbohydrate
C. Steroid
D. Monounsaturated triglyceride
E. Polyunsaturated triglyceride
Steroids
Unlike the phospholipids and fats that we discussed earlier, steroids have a fused ring structure. Although they do not resemble the other lipids, scientists group them with them because they are also hydrophobic and insoluble in water. All steroids have four linked carbon rings and several of them, like cholesterol, have a short tail (Figure 29.11). Many steroids also have the –OH functional group, which puts them in the alcohol classification (sterols).
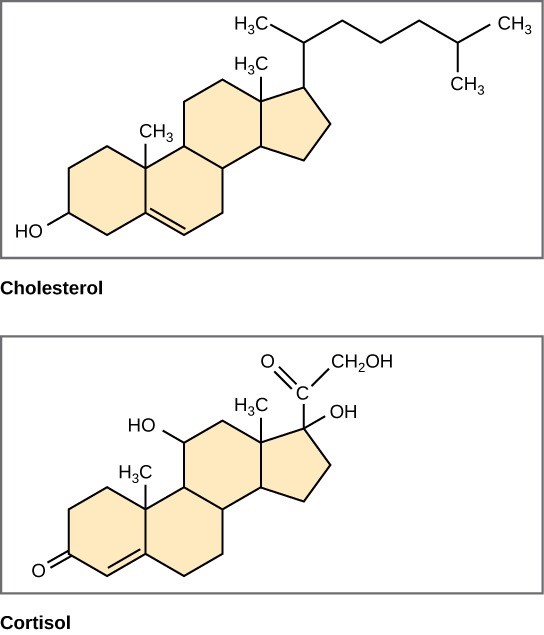
Cholesterol is the most common steroid. The liver synthesizes cholesterol and is the precursor to many steroid hormones such as testosterone and estradiol, which gonads and endocrine glands secrete. It is also the precursor to Vitamin D. Cholesterol is also the precursor of bile salts, which help emulsifying fats and their subsequent absorption by cells. Although lay people often speak negatively about cholesterol, it is necessary for the body’s proper functioning. Sterols (cholesterol in animal cells, phytosterol in plants) are components of the plasma membrane of cells and are found within the phospholipid bilayer.
Reading Question #5
What feature distinguishes a saturated fat from an unsaturated fat?
A. Whether the alignment around double bonds is cis or trans configuration.
B. Whether the bonds between carbons are single or double bonds.
C. Whether the monomers are bound together with ester bonds or glycosidic linkages.
D. The number of fatty acids attached to the glycerol.
Acknowledgements
Adapted from Clark, M.A., Douglas, M., and Choi, J. (2018). Biology 2e. OpenStax. Retrieved from https://openstax.org/books/biology-2e/pages/1-introduction