15 Chapter 15: Species Interactions
Anastasia Chouvalova and Lisa Limeri
Learning Objectives
By the end of this section, students will be able to:
- Explain the predictions and assumptions of the Lotka-Volterra model of predator-prey dynamics.
- Distinguish between symbiotic and non-symbiotic relationships and obligate vs. facultative mutualisms
- Describe and distinguish between species interactions, including the impact (positive/negative/neutral) on each interacting partner: competition, commensalism, amensalism, mutualism, consumption, parasitism.
Consumption: Predation and Herbivory
Predation is a biological interaction where one organism, the predator, kills and eats another organism, its prey. It is distinct from scavenging on dead prey, though many predators also scavenge. Predation and herbivory overlap because seed predators and destructive frugivores kill their “prey.” The concept of predation is broad, defined differently in different contexts, and includes a wide variety of feeding methods
Predation
Perhaps the classical example of species interaction is predation: the consumption of prey by its predator. Nature shows on television highlight the drama of one living organism killing another. Predators are adapted and often highly specialized for hunting, with acute senses such as vision, hearing, or smell. Many predatory animals, both vertebrate and invertebrate, have sharp claws or jaws to grip, kill, and cut up their prey. Other adaptations include stealth and aggressive mimicry that improve hunting efficiency. When prey is detected, the predator assesses whether to attack it. Predators may actively search for or pursue prey (pursuit predation) or sit and wait for prey (ambush predation), often concealed, prior to attack. If the attack is successful, the predator kills the prey, removes any inedible parts like the shell or spines, and eats it.
Herbivory
Herbivory is a form of consumption in which an organism principally eats autotrophs such as plants, algae and photosynthesizing bacteria. More generally, organisms that feed on autotrophs are known as primary consumers. Herbivory is usually limited to animals that eat plants. Fungi, bacteria, and protists that feed on living plants are usually termed plant pathogens (plant diseases), while fungi and microbes that feed on dead plants are described as saprotrophs. Flowering plants that obtain nutrition from other living plants are usually termed parasitic plants.
Two herbivore feeding strategies are grazing (e.g. cows) and browsing (e.g. moose). For a terrestrial mammal to be called a grazer, at least 90% of the forage has to be grass, and for a browser at least 90% tree leaves and twigs. An intermediate feeding strategy is called “mixed-feeding”. In their daily need to take up energy from forage, herbivores of different body masses may be selective in choosing their food. “Selective” means that herbivores may choose their forage source depending on environmental conditions such as season or food availability, but also that they may choose high-quality (and consequently highly nutritious) forage before lower quality. The latter especially is determined by the body mass of the herbivore, with small herbivores selecting for high-quality forage, and with increasing body mass animals are less selective.
Defense Mechanisms against Predation and Herbivory
Predation has a powerful selective effect on prey, and prey evolve anti-predator adaptations such as warning coloration, alarm and other calls, camouflage, copying (mimicry) of well-defended species, and defensive spines and chemicals. Sometimes predator and prey find themselves in an evolutionary arms race, a cycle of adaptations and counter-adaptations. Unlike animals, most plants cannot outrun predators. However, plants have evolved a variety of mechanisms to defend against herbivory. Other species have developed mutualistic relationships; for example, herbivory provides a mechanism of seed distribution that aids in plant reproduction.
The study of communities must consider evolutionary forces that act on the members of the various populations contained within it. Species are not static, but slowly changing and adapting to their environment by natural selection and other evolutionary forces. Species have evolved numerous mechanisms to escape predation and herbivory. These defenses may be mechanical, chemical, physical, or behavioral.
Mechanical defenses, such as the presence of thorns on plants or the hard shell on turtles, discourage animal predation and herbivory by causing physical pain to the predator or by physically preventing the predator from being able to eat the prey. Chemical defenses are produced by many animals as well as plants, such as the foxglove which is extremely toxic when eaten. Figure 15.1 shows some organisms’ defenses against predation and herbivory.
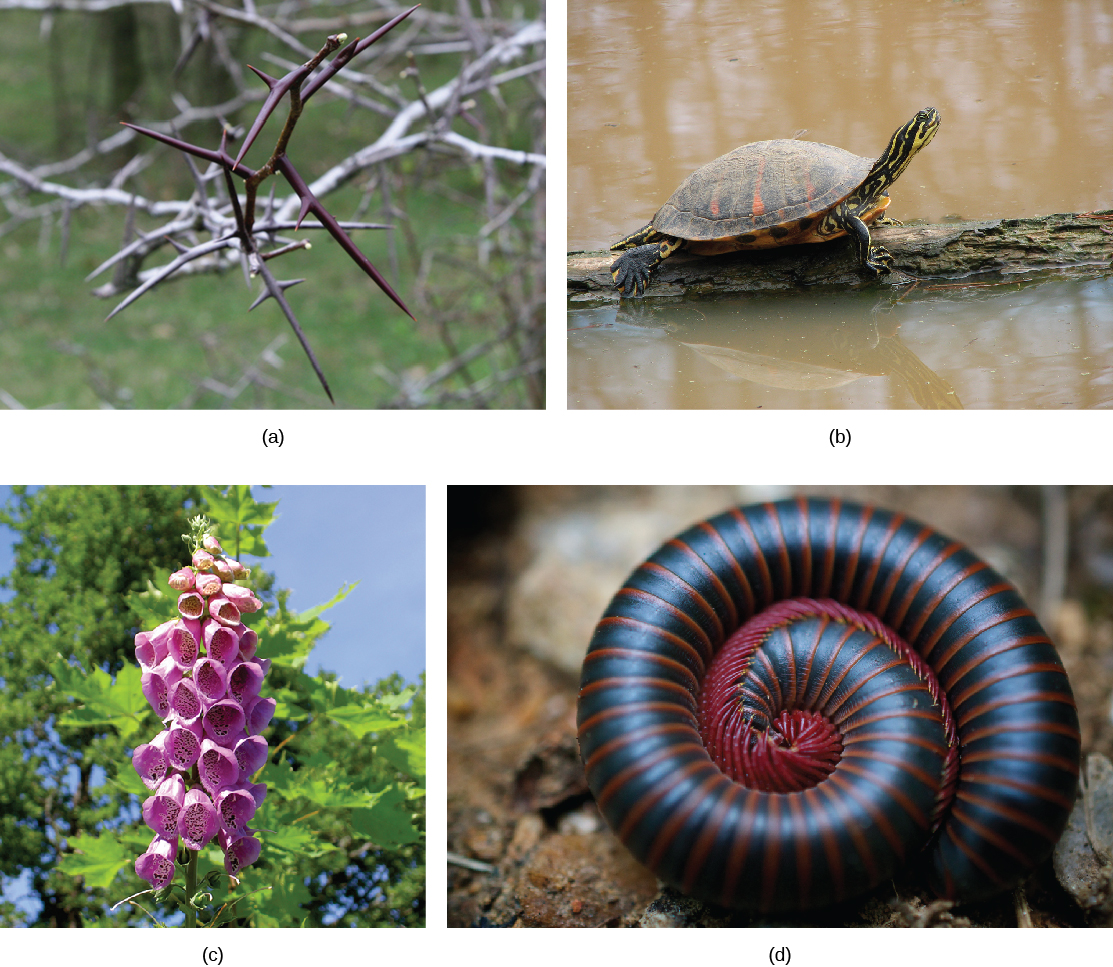
Many species use physical appearance, such as body shape and coloration, to avoid being detected by predators. The tropical walking stick is an insect with the coloration and body shape of a twig which makes it very hard to see when stationary against a background of real twigs (Figure 15.2a). In another example, the chameleon can, within limitations, change its color to match its surroundings (Figure 15.2b). Both of these are examples of camouflage, or avoiding detection by blending in with the background. There are many behavioral adaptations to avoid or confuse predators. Playing dead and traveling in large groups, like schools of fish or flocks of birds, are both behaviors that reduce the risk of being eaten.
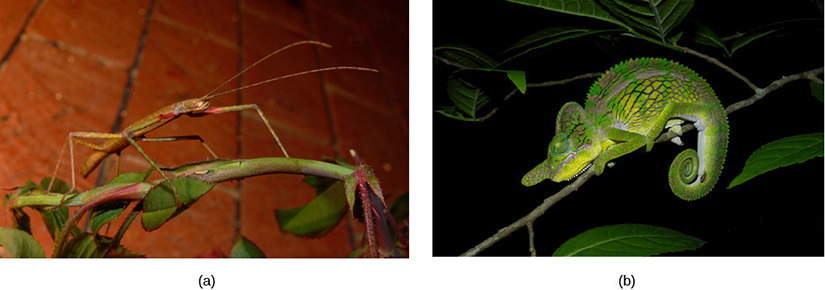
Some species use coloration as a way of warning predators that they are not good to eat. For example, the cinnabar moth caterpillar, the fire-bellied toad, and many species of beetle have bright colors that warn of a foul taste, the presence of toxic chemicals, and/or the ability to sting or bite, respectively. Predators that ignore this coloration and eat the organisms will experience their unpleasant taste or presence of toxic chemicals and learn not to eat them in the future. This type of defensive mechanism is called aposematic coloration, or warning coloration (Figure 15.3).
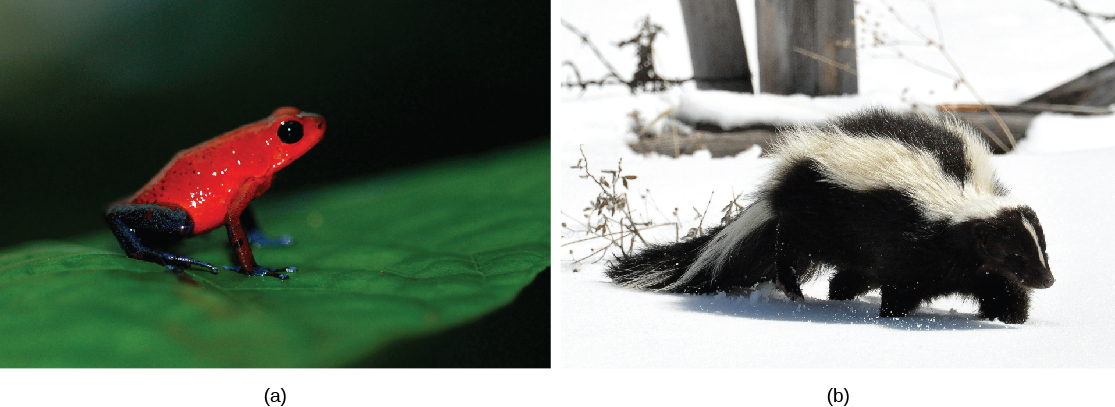
While some predators learn to avoid eating certain potential prey because of their coloration, other species have evolved mechanisms to mimic this coloration to avoid being eaten, even though they themselves may not be unpleasant to eat or contain toxic chemicals. In Batesian mimicry, a harmless species imitates the warning coloration of a harmful one. Assuming they share the same predators, this coloration then protects the harmless ones, even though they do not have the same level of physical or chemical defenses against predation as the organism they mimic. Many insect species mimic the coloration of wasps or bees, which are stinging, venomous insects, thereby discouraging predation (Figure 15.4).
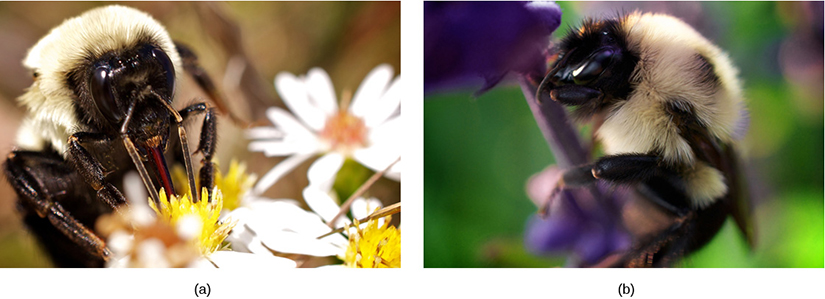
In Müllerian mimicry, multiple species share the same warning coloration, but all of them actually have defenses. Figure 15.5 shows a variety of foul-tasting butterflies with similar coloration.
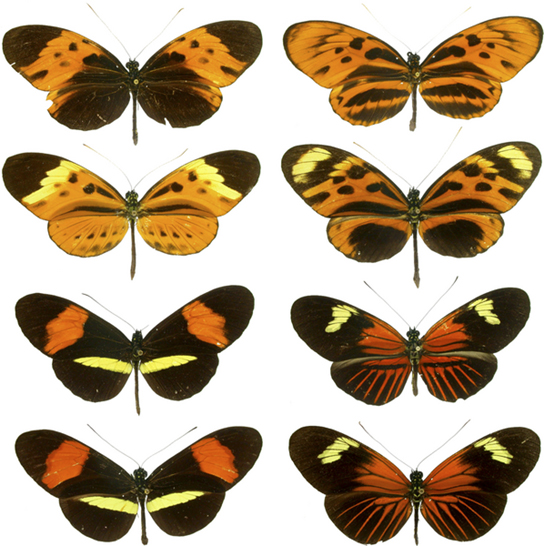
Link to Learning
Go to this website to see stunning examples of mimicry.
Modeling Predator/Prey Dynamics
Population sizes of predators and prey in a community are not constant over time: in most cases, they vary in cycles that appear to be related. The most often cited example of predator-prey dynamics is seen in the cycling of the lynx (predator) and the snowshoe hare (prey), using nearly 200 year-old trapping data from North American forests (Figure 15.6). Lotka and Volterra independently proposed in the 1920s a mathematical model for the population dynamics of a predator and prey that would explain this observation. These Lotka-Volterra predator-prey equations have since become an iconic model of mathematical biology. The Lotka-Volterra model predicts that as the hare numbers increase, there is more food available for the lynx, allowing the lynx population to increase as well. When the lynx population grows to a threshold level, however, they kill so many hares that hare population begins to decline, followed by a decline in the lynx population because of scarcity of food. When the lynx population is low, the hare population size begins to increase due to low predation pressure, starting the cycle anew. This cycle of predator and prey lasts approximately 10 years, with the predator population lagging 1–2 years behind that of the prey population.
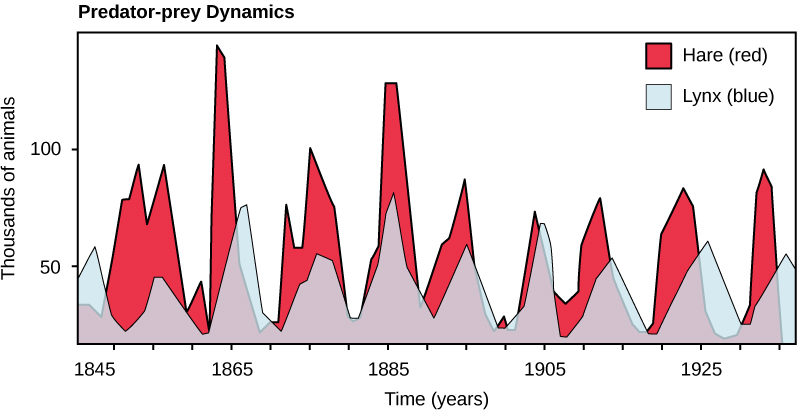
Link to Learning
The Lotka-Volterra model equations are outside of the scope of this course, but you can find more information on them at this link: https://math.libretexts.org/Bookshelves/Applied_Mathematics/Mathematical_Biology_(Chasnov)/01%3A_Population_Dynamics/1.04%3A_The_Lotka-Volterra_Predator-Prey_Model
Like all mathematical models, the Lotka-Volterra model of predator-prey dynamics makes a number of assumptions that must be met in order for the model’s predictions to be observed. These assumptions include that the number of prey grow exponentially in the absence of predators (there is unlimited food available to the prey), and that the number of predators decay exponentially in the absence of prey (predators must eat prey or starve). Contact between predators and prey increases the number of predators and decreases the number of prey.
The Lotka-Volterra model is one of the earliest predator-prey models to be based on sound mathematical principles. It forms the basis of many models used today in the analysis of population dynamics. Unfortunately, in its original form Lotka-Volterra has some significant problems. It predicts that there is no stable equilibrium point, but rather that the predator and prey populations will cycle endlessly. While this cycling has been observed in some situations in nature (such as the historical trapping data from lynxes and hares), it is not very common.
The Lotka-Volterra by itself is not sufficient to model many predator-prey systems because it assumes that the only factors influencing predator and prey populations are the population sizes of the predators and prey. However, there are other density-dependent factors that are important in influencing prey population size, in addition to predation. One possibility is that the cycling is inherent in the hare population due to density-dependent effects such as lower fecundity (maternal stress) caused by crowding when the hare population gets too dense. The hare cycling would then induce the cycling of the lynx because it is the lynxes’ major food source. The more we study communities, the more complexities we find, allowing ecologists to derive more accurate and sophisticated models of population dynamics.
Reading Question #1
What is the primary prediction of the Lotka-Volterra models?
A. Predator population sizes will always be higher than prey population sizes.
B. Predator and prey population sizes do not affect each other.
C. Predator and prey population sizes cyclically influence each other.
D. Predators influence prey population sizes but prey do not influence predator’s population sizes.
Parasitism
Parasitism is a symbiotic relationship between species, where one organism, the parasite, lives on or inside another organism, the host, causing it some harm, and is adapted structurally to this way of life. Like predation, parasitism is a type of consumer-resource interaction, but unlike predators, parasites are typically much smaller than their hosts, do not directly kill them, and often live in or on their hosts for an extended period. Parasites of animals are highly specialized, and reproduce at a faster rate than their hosts. Classic examples include interactions between vertebrate hosts and tapeworms, flukes, the malaria-causing Plasmodium species, and fleas.
Parasitism describes a relationship in which one member of the association benefits at the expense of the other. So, while the parasite itself benefits, the host organism is harmed. The parasite typically weakens the host as it consumes resources that the host would normally benefit from. The parasite usually does not directly kill the host, but could indirectly contribute to the host’s death by weakening it and robbing it of resources.
Parasites are classified in a variety of overlapping categories, based on their interactions with their hosts and on their life-cycles, which are sometimes very complex. An obligate parasite depends completely on the host to complete its life cycle and cannot survive without the host. In contrast, a facultative parasite benefits from parasitizing from a host but does not require its host for survival. An endoparasite lives inside the host’s body; an ectoparasite lives outside, on the host’s surface. Mesoparasites are in between endoparasites and ectoparasites—they enter an opening in the host’s body and remain partly embedded there. Some parasites can be generalists, feeding on a wide range of hosts, but many parasites are specialists and extremely host-specific.
The reproductive cycles of parasites are often very complex, sometimes requiring more than one host species. A tapeworm is a parasite that causes disease in humans when contaminated, undercooked meat is consumed. The tapeworm can live inside the intestine of the host for several years, benefiting from the food the host is eating, and may grow to be over 50 ft long by adding segments. The parasite moves from species to species in a cycle, making two hosts necessary to complete its life cycle.
Many parasites that has multiple hosts are trophically-transmitted between their hosts. Trophically-transmitted parasites are transmitted by being eaten by a host. In their juvenile stages they infect a host termed the intermediate host. The intermediate host is a host the parasite can infect, but cannot complete its reproduction inside of. When the intermediate-host animal is eaten by a predator, the definitive host, the parasite survives the digestion process and matures into an adult; some live as intestinal parasites. When parasites have multiple hosts, the definitive host is the host that they can reproduce inside of. Many trophically-transmitted parasites modify the behavior of their intermediate hosts, increasing their chances of being eaten by a predator.
Connection to Evolution
Malaria is a parasitic disease in humans that is transmitted by infected female mosquitoes, including Anopheles gambiae (Figure 15.7a), and is characterized by cyclic high fevers, chills, flu-like symptoms, and severe anemia. Plasmodium falciparum and P. vivax are the most common causative agents of malaria, and P. falciparum is the most deadly (Figure 15.7b). When promptly and correctly treated, P. falciparum malaria has a mortality rate of 0.1%. However, in some parts of the world, the parasite has evolved resistance to commonly used malaria treatments, so the most effective malarial treatments can vary by geographic region.
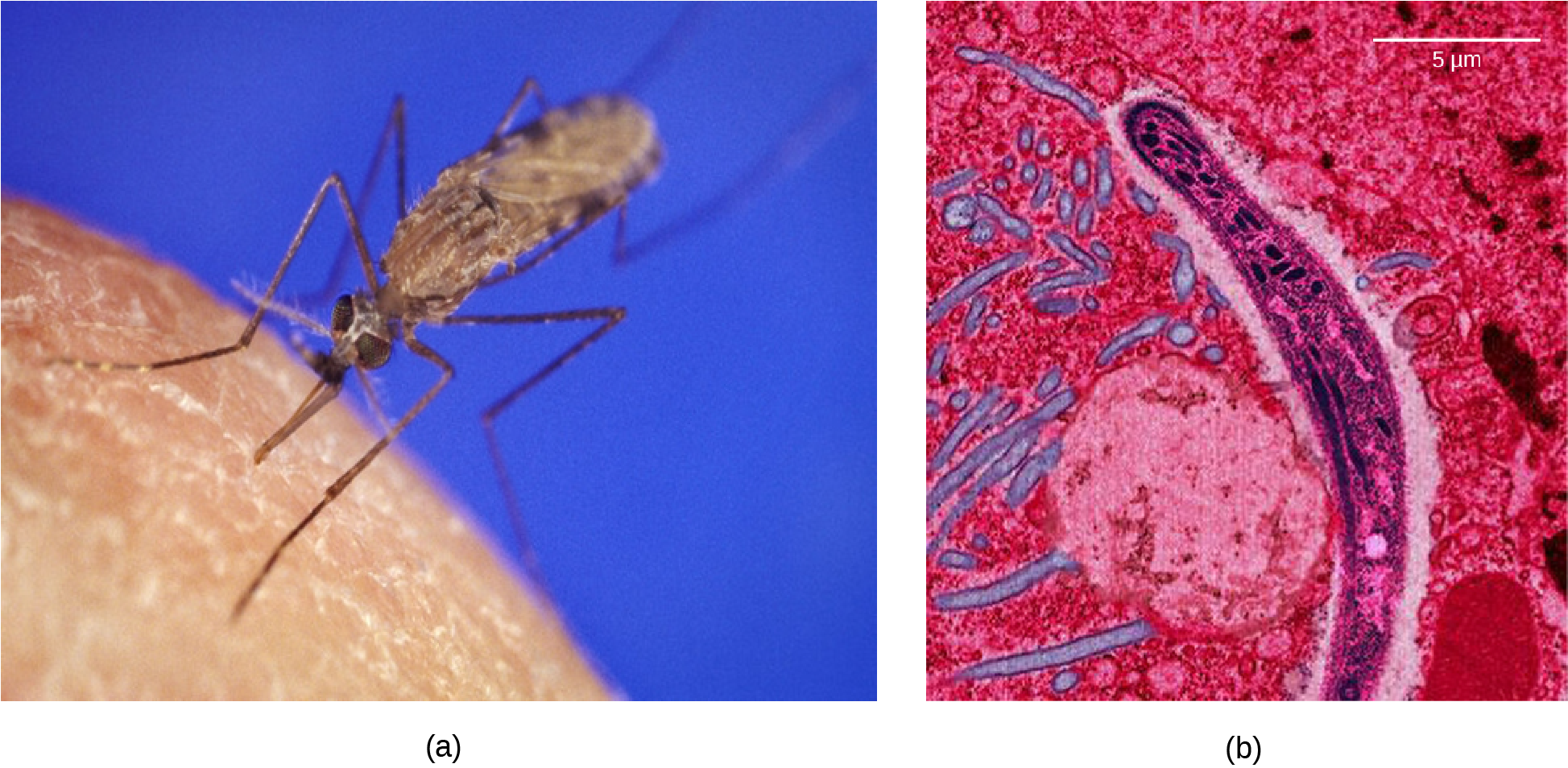
In Southeast Asia, Africa, and South America, P. falciparum has developed resistance to the anti-malarial drugs chloroquine, mefloquine, and sulfadoxine-pyrimethamine. P. falciparum, which is haploid during the life stage in which it is infectious to humans, has evolved multiple drug-resistant mutant alleles of the dhps gene. Varying degrees of sulfadoxine resistance are associated with each of these alleles. Being haploid, P. falciparum needs only one drug-resistant allele to express this trait.
In Southeast Asia, different sulfadoxine-resistant alleles of the dhps gene are localized to different geographic regions. This is a common evolutionary phenomenon that occurs because drug-resistant mutants arise in a population and interbreed with other P. falciparum isolates in close proximity. Sulfadoxine-resistant parasites cause considerable human hardship in regions where this drug is widely used as an over-the-counter malaria remedy. As is common with pathogens that multiply to large numbers within an infection cycle, P. falciparum evolves relatively rapidly (over a decade or so) in response to the selective pressure of commonly used anti-malarial drugs. For this reason, scientists must constantly work to develop new drugs or drug combinations to combat the worldwide malaria burden (Summit Vinayak et al., 2010).
Sumiti Vinayak, et al., “Origin and Evolution of Sulfadoxine Resistant Plasmodium falciparum,” Public Library of Science Pathogens 6, no. 3 (2010): e1000830, doi:10.1371/journal.ppat.1000830.
Variations on parasitism
Classic parasites live in or on the host’s body. However, there are many interactions also described as parasitism where the parasite lives in close relationship with the host, but not directly in or on it.
Social parasitism
Social parasites take advantage of interspecific interactions between members of eusocial animals such as ants, termites, and bumblebees. An example is the large blue butterfly, Phengaris arion. Larvae (caterpillars) of this species mimic ants by emitting ant queen pheromones. Ant workers find larvae and care for them as they would their queen; they take it to an inner chamber in their nest and feed it. Another exampe is a bumblebee, Bombus bohemicus, which invades the hives of other bees and takes over reproduction while their young are raised by host workers.
Brood Parasitism
In brood parasitism, the parasite lays their eggs inside the nest of another species; the hosts act as parents as they raise the young as their own (fig 15.8). Brood parasites include birds in different families such as cowbirds, whydahs, cuckoos, and black-headed ducks. These birds do not build nests of their own, but leave their eggs in nests of other species. The eggs of some brood parasites mimic those of their hosts, while some cowbird eggs have tough shells, making them hard for the hosts to kill by piercing. The adult female European cuckoo further mimics a predator, the European sparrowhawk, giving her time to lay her eggs in the host’s nest unobserved.
Parasitoidism
Some relationships that result in the prey’s death are not generally called predation. A parasitoid, such as an ichneumon wasp, lays its eggs in or on its host; the eggs hatch into larvae, which eat the host, and it inevitably dies. This is distinct from classic parasitism because parasites do not directly kill their hosts. A predator can be defined to differ from a parasitoid in that it has many prey, captured over its lifetime, where a parasitoid’s larva has just one, or at least has its food supply provisioned for it on just one occasion.
Most parasitoids are parasitoid wasps or other hymenopterans. They can be divided into two groups, idiobionts and koinobionts, differing in their treatment of their hosts.
Reading Question #2
A wasp lays its eggs in a caterpillar. For a while, the caterpillar is unaffected and grows normally. The wasp eggs hatch inside the caterpillar and when the wasp larvae reach a specific developmental stage, they consume the caterpillar from the inside out and burst from the caterpillar’s body, killing it. This scenario best describes which of the following species interactions?
A. Predation
B. Herbivory
C. Parastism
D. Parasitoidism
E. Commensalism
Symbiosis
Symbiotic relationships are close interactions between individuals of different species over an extended period of time which impact the abundance and distribution of the associating populations. Symbioses can include relationships where one partner benefits and the other is unaffected (commensalism), one partner benefits while the other is harmed (parasitism), or both partners benefit (mutualism).
Commensalism
A commensal relationship occurs when one species benefits from the interaction while the other neither benefits nor is harmed. Birds nesting in trees provide an example of a commensal relationship (Fig 15.9). The tree is not harmed by the presence of the nest among its branches. The nests are light and produce little strain on the structural integrity of the branch, and most of the leaves, which the tree uses to get energy by photosynthesis, are above the nest so they are unaffected. The bird, on the other hand, benefits greatly. If the bird had to nest in the open, its eggs and young would be vulnerable to predators. Another example of a commensal relationship is the pilot fish and the shark. The pilot fish feed on the leftovers of the host’s meals, and the host is not affected in any way.
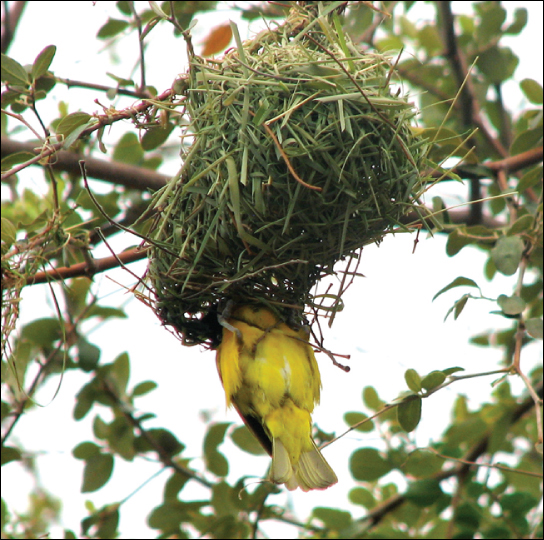
Reading Question #3
Which of the following is the best example of commensalism?
A. A nematode is ingested by a human and attacks the intestinal lining in order to obtain nourishment.
B. An oxpecker (a type of bird) lands on a rhinoceros and eats parasites such as ticks from its body, getting nourishment in the process.
C. Barnacles settle on the body of a large whale, who remains undisturbed.
D. A shrimp constructs a burrow for goby fish and the fish defends the shrimp from higher-level consumers.
Mutualism
A mutualism is a symbiotic relationship where two species benefit from their interaction. For example, termites have a mutualistic relationship with protozoa that live in the insect’s gut (Fig 15.10a). The termite benefits from the ability of bacterial symbionts within the protozoa to digest cellulose. The termite itself cannot do this, and without the protozoa, it would not be able to obtain energy from its food (cellulose from the wood it chews and eats). The protozoa and the bacterial symbionts benefit by having a protective environment and a constant supply of food from the wood chewing actions of the termite. Lichens are not an individual organism, but a mutualistic relationship between fungus and photosynthetic algae or bacteria (Fig 15.10b). As these symbionts grow together, the glucose produced by the algae provides nourishment for both organisms, whereas the physical structure of the lichen protects the algae from the elements and makes certain nutrients in the atmosphere more available to the algae.
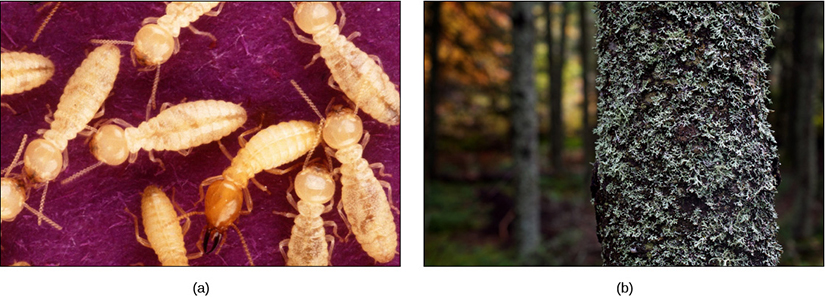
Fungus/Plant Mutualism
One of the most remarkable mutualisms in all of nature is the relationship between vascular plants and the fungus, mycorrhizae. Mycorrhiza, which is derived from the Greek words myco meaning fungus and rhizo meaning root, refers to the fungal partner of a mutualistic association between vascular plant roots and their symbiotic fungi. Nearly 90% of all vascular plant species have mycorrhizal partners. In a mycorrhizal association, the fungal mycelia use their extensive network of hyphae and large surface area in contact with the soil to channel water and minerals from the soil into the plant. In exchange, the plant supplies the products of photosynthesis to fuel the metabolism of the fungus.
There are several basic types of mycorrhizae. Ectomycorrhizae (“outside” mycorrhizae) depend on fungi enveloping the roots in a sheath (called a mantle). Hyphae grow from the mantle into the root and envelope the outer layers of the root cells in a network of hyphae (Fig. 15.11). The fungal partner can belong to the Ascomycota, Basidiomycota or Zygomycota. Endomycorrhizae (“inside” mycorrhizae), also called arbuscular mycorrhizae, are produced when the fungi grow inside the root in a branched structure called an arbuscule (from the Latin for “little trees”). The fungal arbuscules penetrate root cells between the cell wall and the plasma membrane and are the site of the metabolic exchanges between the fungus and the host plant (Fig 15.11). Orchids rely on a third type of mycorrhiza. Orchids are epiphytes that typically produce very small airborne seeds without much storage to sustain germination and growth. Their seeds will not germinate without a mycorrhizal partner. After nutrients in the seed are depleted, fungal symbionts support the growth of the orchid by providing necessary carbohydrates and minerals. Some orchids continue to be mycorrhizal throughout their life cycle.
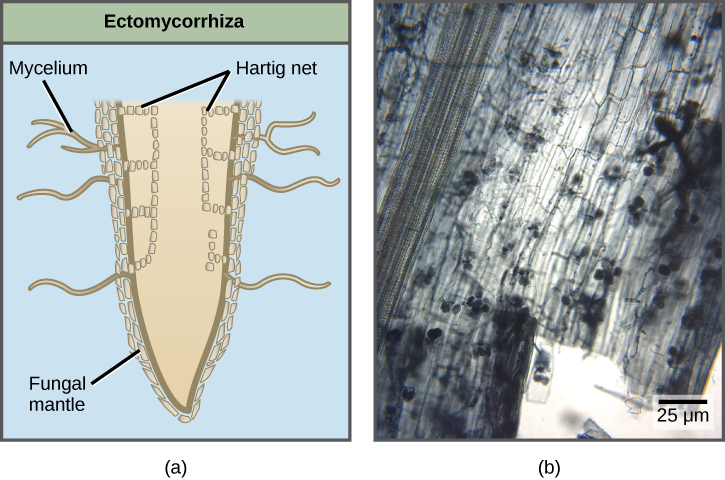
Some plants are able to survive without an mycorrhizal partner, but they do better when they are benefitting from a mutualistic relationship with mycorrhizae. These plants are an example of a facultative mutualism, where the mutualism is beneficial, but partners are capable of surviving without the other. In contrast, other plants are completely unable to survive without mycorrhizae. These plants are an example of an obligate mutualism, a relationship where one or both partners requires the partnership to survive.
Other examples of fungus–plant mutualism include the endophytes: fungi that live inside tissue without damaging the host plant. Endophytes release toxins that repel herbivores, or confer resistance to environmental stress factors, such as infection by microorganisms, drought, or heavy metals in soil.
Evolution Connection: Coevolution of Land Plants and Mycorrhizae
As we have seen, mycorrhizae are the fungal partners of a mutually beneficial symbiotic association that coevolved between roots of vascular plants and fungi. A well-supported theory proposes that fungi were instrumental in the evolution of the root system in plants and contributed to the success of Angiosperms. The bryophytes (mosses and liverworts), which are considered the most ancestral plants and the first to survive and adapt on land, have simple underground rhizoids, rather than a true root system, and therefore cannot survive in dry areas. However, some bryophytes have arbuscular mycorrhizae and some do not.
True roots first appeared in the ancestral vascular plants: Vascular plants that developed a system of thin extensions from their roots would have had a selective advantage over nonvascular plants because they had a greater surface area of contact with the fungal partners than did the rhizoids of mosses and liverworts. The first true roots would have allowed vascular plants to obtain more water and nutrients in the ground.
Fossil records indicate that fungi actually preceded the invasion of ancestral freshwater plants onto dry land. The first association between fungi and photosynthetic organisms on land involved moss-like plants and endophytes. These early associations developed before roots appeared in plants. Slowly, the benefits of the endophyte and rhizoid interactions for both partners led to present-day mycorrhizae: About 90% of today’s vascular plants have associations with fungi in their rhizosphere.
The fungi involved in mycorrhizae display many characteristics of ancestral fungi; they produce simple spores, show little diversification, do not have a sexual reproductive cycle, and cannot live outside of a mycorrhizal association. The plants benefited from the association because mycorrhizae allowed them to move into new habitats and allowed the increased uptake of nutrients, which gave them an enormous selective advantage over plants that did not establish symbiotic relationships.
Reading Question #4
A farmer is experimenting with different methods to get the best yield from their crops. They discover that their soybeans are capable of growing on their own, but when mycorrhizae are introduced, the soybeans grow larger and produce a greater crop yield. The relationship between the soybean and mycorrhizae is best described as:
A. Obligate mutualism
B. Facultative mutualism
C. Commensalism
D. facultative parasitism
Evolution Connection: Endosymbiosis
Symbiosis is a relationship in which organisms from two separate species depend on each other for their survival. Endosymbiosis (endo- = “within”) is a mutually beneficial relationship in which one organism lives inside the other. Endosymbiotic relationships abound in nature. For example, microbes that live in our gut produce vitamin K. This relationship is beneficial for us because we are unable to synthesize vitamin K. It is also beneficial for the microbes because they are protected from other organisms and from drying out, and they receive abundant food from the environment of the large intestine.
Scientists have long noticed that bacteria, mitochondria, and chloroplasts are similar in size. We also know that bacteria have DNA and ribosomes, just like mitochondria and chloroplasts. Scientists believe that host cells and bacteria formed an endosymbiotic relationship when the host cells ingested both aerobic and autotrophic bacteria (cyanobacteria) but did not destroy them. Through many millions of years of evolution, these ingested bacteria became more specialized in their functions, with the aerobic bacteria becoming mitochondria and the autotrophic bacteria becoming chloroplasts.
Fungus/Animal Mutualism
Fungi have evolved mutualisms with numerous arthropods. Arthropods depend on the fungus for protection from predators and pathogens, while the fungus obtains nutrients and a way to disseminate spores into new environments.
One example is the leaf-cutter ants of Central and South America which literally farm fungi. They cut disks of leaves from plants and pile them up in subterranean gardens (Figure 15.12). Fungi are cultivated in these disk gardens, digesting the cellulose in the leaves that the ants cannot break down. Once smaller sugar molecules are produced and consumed by the fungi, the fungi in turn become a meal for the ants. The insects also patrol their garden, preying on competing fungi. Both ants and fungi benefit from this mutualistic association. The fungus receives a steady supply of leaves and freedom from competition, while the ants feed on the fungi they cultivate.
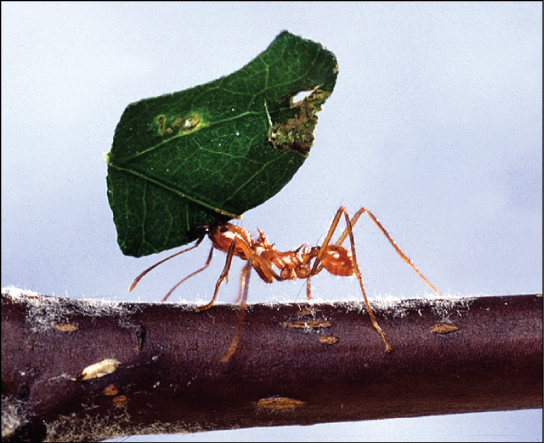
Reading Question #5
Which of the following describes symbiosis?
A. Two species live in close contact with each other.
B. Two species interact in ways that mutually benefit each other.
C. Two species live in the same region and are closely related.
D. Two species use the same resources.
References
Adapted from
Clark, M.A., Douglas, M., and Choi, J. (2018). Biology 2e. OpenStax. Retrieved from https://openstax.org/books/biology-2e/pages/45-6-community-ecology
and
Chasnov (2022). The Lotka-Volterra Predator-Prey Model. LibreTexts. Retrieved from https://math.libretexts.org/Bookshelves/Applied_Mathematics/Mathematical_Biology_(Chasnov)/01%3A_Population_Dynamics/1.04%3A_The_Lotka-Volterra_Predator-Prey_Model
and
Holmberg, T. J. (2023). Antagonistic Interactions. LibreTexts. Retrieved from https://bio.libretexts.org/Sandboxes/tholmberg_at_nwcc.edu/BIOL_1213/04%3A_Unit_4%3A_-_Ecology/4.03%3A_Community_Ecology/4.3.2%3A_Antagonistic_Interactions
and
Various authors. (2022). Ecology for All!. LibreTexts. Retrieved from https://bio.libretexts.org/Courses/Gettysburg_College/01%3A_Ecology_for_All/16%3A_Antagonistic_Interactions/16.04%3A_Parasitism#:~:text=Parasitoids%20are%20insects%20which%20sooner,dipterans%20such%20as%20phorid%20flies.